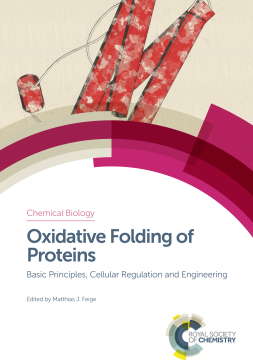
Additional Information
Book Details
Abstract
The formation of disulphide bonds is probably the most influential modification of proteins. These bonds are unique among post-translational modifications of proteins as they can covalently link cysteine residues far apart in the primary sequence of a protein. This has the potential to convey stability to otherwise marginally stable structures of proteins. However, the reactivity of cysteines comes at a price: the potential to form incorrect disulphide bonds, interfere with folding, or even cause aggregation. An elaborate set of cellular machinery exists to catalyze and guide this process: facilitating bond formation, inhibiting unwanted pairings and scrutinizing the outcomes. Only in recent years has it become clear how intimately connected this cellular machinery is with protein folding helpers, organellar redox balance and cellular homeostasis as a whole.
This book comprehensively covers the basic principles of disulphide bond formation in proteins and describes the enzymes involved in the correct oxidative folding of cysteine-containing proteins. The biotechnological and pharmaceutical relevance of proteins, their variants and synthetic replicates is continuously increasing. Consequently this book is an invaluable resource for protein chemists involved in realted research and production.
Matthias J. Feige studied biochemistry at the Swiss Federal Institute of Technology (ETH Zurich) and TUM. In 2009, he obtained his PhD in biochemistry under the supervision of Johannes Buchner at the TUM. As a postdoctoral fellow in the laboratory of Linda Hendershot at the St. Jude Children’s Research Hospital, Memphis, TN, USA, he expanded his research towards cell biology and since 2015 heads the laboratory for cellular protein biochemistry at the TUM. Matthias J Feige’s laboratory aims at understanding how cells control and maintain the integrity of their proteome. He is particularly interested in proteins of the secretory pathway - proteins that are ultimately secreted or localized on the cell surface and allow cells to interact with their environment. Using an interdisciplinary approach from protein biochemistry to cell biology he analyses the machinery and mechanisms that monitor cellular protein biogenesis. By focusing on proteins of immunological and biomedical relevance, he seeks a molecular understanding of fundamental biological processes that at the same time may help in developing new approaches for protein engineering and human therapy.
Table of Contents
Section Title | Page | Action | Price |
---|---|---|---|
Cover\r | Cover | ||
Oxidative Folding of Proteins: Basic Principles, Cellular Regulation and Engineering | i | ||
Foreword | v | ||
Preface | vii | ||
Contents | ix | ||
Section I - Principles and Analysis of Disulfide Bond Formation | 1 | ||
Chapter 1.1 - Disulfide Bonds in Protein Folding and Stability | 3 | ||
1.1.1 Stabilization of Proteins by Disulfide Bonds | 3 | ||
1.1.2 Disulfide Bonds in Protein Folding Reactions: Biophysical Considerations | 8 | ||
1.1.3 Distinctions Between In vitro Refolding Assays and Protein Biosynthesis in a Cell | 11 | ||
1.1.4 Disulfide Bonds in ER Protein Folding | 14 | ||
1.1.5 Formation of Disulfide Bonds Between Sequential Cysteines | 16 | ||
1.1.6 Disulfide Bonds Between Non-sequential, Often Long-range, Cysteines | 17 | ||
1.1.7 Non-native Disulfide Bonds as a Prerequisite to Correct Protein Maturation | 19 | ||
1.1.8 Disulfide Bonds, Protein Misfolding and Human Disease | 20 | ||
1.1.9 Concluding Thoughts | 24 | ||
Acknowledgements | 25 | ||
References | 25 | ||
Chapter 1.2 - Techniques to Monitor Disulfide Bond Formation and the Reduction Potential of Cysteine–Cystine Couples In vitro and In vivo | 34 | ||
1.2.1 Introduction | 34 | ||
1.2.2 Examples of Biological Cysteine–Cystine Couples | 37 | ||
1.2.3 Determination of Standard Reduction Potentials | 39 | ||
1.2.4 In situ Cysteine–Cystine Distributions | 41 | ||
1.2.5 Genetically Encoded Fluorescent Protein Sensors to Monitor Intracellular Redox Couples | 43 | ||
1.2.5.1 General Principles | 43 | ||
1.2.5.2 roGFP-based Sensors for the Monitoring of EGSH | 44 | ||
1.2.6 EGSH Dynamics in Multicellular Organisms | 45 | ||
1.2.7 Conclusions and Perspectives | 46 | ||
References | 47 | ||
Chapter 1.3 - Real-time Detection of Thiol Chemistry in Single Proteins | 52 | ||
1.3.1 Introduction | 52 | ||
1.3.1.1 Single-molecule Pulling Instruments | 53 | ||
1.3.1.2 Mechanical Fingerprints Provide Unambiguous Identification of Single-protein Tethers | 55 | ||
1.3.2 Mechanochemical Cleavage of Cryptic Protein Disulfide Bonds | 56 | ||
1.3.2.1 Disulfide Cleavage with Low Molecular Weight Thiols | 59 | ||
1.3.2.2 Two Pathways for Disulfide Cleavage by the Hydroxyl Ion | 60 | ||
1.3.2.3 Rules for Single-protein Thiol Chemistry Experiments | 61 | ||
1.3.3 Enzymatic Cleavage of Protein Disulfide Bonds | 61 | ||
1.3.3.1 Single-molecule Reduction by Thioredoxins | 61 | ||
1.3.3.2 Prokaryotic and Eukaryotic Thioredoxins Demonstrate Alternative Mechanisms | 63 | ||
1.3.4 Inferring Molecular Pathways of Oxidative Folding | 64 | ||
1.3.4.1 A Single-molecule Assay for Oxidative Folding by Protein Disulfide Isomerase | 64 | ||
1.3.4.2 DsbA is a High-efficiency Bacterial Oxidoreductase | 68 | ||
1.3.4.3 Non-enzymatic Oxidative Folding | 69 | ||
1.3.5 Conformational Changes and Allostery Through Cysteine Redox and Disulfide Isomerization | 70 | ||
1.3.5.1 Disulfide Isomerization in Superoxide Dismutase 1 (SOD1) | 71 | ||
1.3.5.2 S-Glutathionylation of Cryptic Thiols Regulates Muscle Elasticity | 72 | ||
1.3.5.3 A Protein Thioester Responsible for Bacterial Adhesion | 72 | ||
1.3.6 Conclusion | 76 | ||
References | 77 | ||
Chapter 1.4 - Analysis of Disulfide Bond Formation in Therapeutic Proteins | 81 | ||
1.4.1 Introduction | 81 | ||
1.4.2 Mechanisms of Disulfide Bond Misassembly and Degradation | 82 | ||
1.4.2.1 Cleavage of Intra- and Interchain Disulfide Bonds | 83 | ||
1.4.2.2 Disulfide Bond Scrambling | 84 | ||
1.4.2.3 β-Elimination | 84 | ||
1.4.2.4 Trisulfide Bond Formation | 86 | ||
1.4.2.5 Oxidation, Cysteinylation and Glutathionylation | 87 | ||
1.4.3 Analytical Methods | 87 | ||
1.4.3.1 Electrophoretic Methods | 88 | ||
1.4.3.2 Chemical Labeling and Spectroscopic Detection | 88 | ||
1.4.3.3 Mass Spectrometry-based Methods | 90 | ||
1.4.3.3.1 MS Sample Preparation Strategies | 90 | ||
1.4.3.3.2 LC-MS-based Peptide Profile Comparison | 91 | ||
1.4.3.3.3 Partial Reduction and Specific Alkylation | 93 | ||
1.4.3.3.4 Fragmentation of Disulfide-linked Peptides | 93 | ||
1.4.3.3.5 In-source Reduction of Disulfide Bonds | 94 | ||
1.4.3.3.6 Stable Isotope Labeling of Disulfide Bonded Peptides | 94 | ||
1.4.4 Conclusion and Outlook | 95 | ||
References | 96 | ||
Section II - Disulfide Bonds in Peptides and Proteins: Structure, Function and Evolution | 99 | ||
Chapter 2.1 - Evolutionary Adaptations to Cysteine-rich Peptide Folding | 101 | ||
2.1.1 Introduction | 101 | ||
2.1.1.1 Cysteine-rich Peptides – a Large and Diverse Family | 101 | ||
2.1.1.2 Scope of the Chapter: Evolutionary Adaptations to Conopeptide Folding | 104 | ||
2.1.2 Conopeptide Folding – a Case Study | 105 | ||
2.1.2.1 Conopeptides as Models for the Study of Cysteine-rich Peptide Folding | 105 | ||
2.1.2.2 Conopeptide Size and Cysteine Content | 105 | ||
2.1.2.3 Conopeptide Sequence and Structural Diversity | 107 | ||
2.1.2.4 Overview of In vitro Challenges with Conopeptide Folding | 110 | ||
2.1.3 Adaptations to Conopeptide Folding | 110 | ||
2.1.3.1 The Role of the Propeptide in Conopeptide Folding | 110 | ||
2.1.3.2 Post-translational Modifications | 113 | ||
2.1.3.3 Molecular Chaperones and Folding Enzymes | 114 | ||
2.1.3.3.1 Peptidylprolyl Isomerases (PPIases) | 115 | ||
2.1.3.3.2 BiP | 116 | ||
2.1.3.3.3 PDI | 117 | ||
2.1.3.3.4 csPDI | 118 | ||
2.1.3.3.4.1\rReoxidation of PDI/csPDI.Upon oxidation of a substrate protein, members of the PDI family need to be reoxidized to regain cataly... | 120 | ||
2.1.4 Conclusions and Outlook | 121 | ||
Acknowledgements | 122 | ||
References | 122 | ||
Chapter 2.2 - In vitro Refolding of Proteins | 129 | ||
2.2.1 Introduction | 129 | ||
2.2.2 Inclusion Bodies (IBs) | 130 | ||
2.2.3 IB Preparation and Purification | 132 | ||
2.2.4 Protein Refolding | 134 | ||
2.2.4.1 Refolding Methods | 134 | ||
2.2.4.1.1 Rapid Dilution | 134 | ||
2.2.4.1.2 Dialysis | 136 | ||
2.2.4.1.3 Matrix-assisted Refolding | 136 | ||
2.2.4.2 The Multi-parameter Space of Protein Refolding | 138 | ||
2.2.4.3 Formation of Correct Disulfide Bonds | 142 | ||
2.2.5 The REFOLD Database | 143 | ||
2.2.6 Screening for Suitable Refolding Conditions | 143 | ||
2.2.7 Screening for Suitable Refolding Conditions Using Metal Chelate Chromatography | 145 | ||
2.2.8 Activity Assays – the Heart of a Successful Refolding Screen | 146 | ||
2.2.9 Conclusion | 147 | ||
Acknowledgements | 147 | ||
References | 147 | ||
Chapter 2.3 - Allosteric Disulfide Bonds | 152 | ||
2.3.1 Introduction | 152 | ||
2.3.2 Evolution of Disulfide Bonds | 153 | ||
2.3.3 Classification of Disulfide Bonds | 153 | ||
2.3.4 Emerging Allosteric Configurations | 156 | ||
2.3.5 Cleavage of Allosteric Disulfide Bonds | 158 | ||
2.3.6 Processes That Are Controlled by Allosteric Disulfide Bonds | 160 | ||
2.3.6.1 Hemostasis | 160 | ||
2.3.6.1.1 Platelet Adhesion | 160 | ||
2.3.6.1.2 Blood Coagulation | 162 | ||
2.3.6.2 Immune Response | 164 | ||
2.3.6.3 Viral Entry | 166 | ||
2.3.7 Targeting Disulfide Bonds | 167 | ||
2.3.8 Future Perspectives | 168 | ||
References | 168 | ||
Section III - Oxidative Folding in the Cell | 175 | ||
Chapter 3.1 - Disulfide Bond Formation and Isomerization in Escherichia coli | 177 | ||
3.1.1 Introduction | 177 | ||
3.1.2 Disulfide Bond Formation | 178 | ||
3.1.2.1 The Periplasmic Dithiol Oxidase DsbA | 178 | ||
3.1.2.2 DsbB | 181 | ||
3.1.2.2.1 Regeneration of Oxidized DsbA | 181 | ||
3.1.2.2.2 Structure and Mechanism of DsbB | 182 | ||
3.1.3 Disulfide Bond Isomerization | 183 | ||
3.1.3.1 DsbC, a Thioredoxin-like Homodimer with Disulfide Isomerase and Disulfide Reductase Activity | 183 | ||
3.1.3.2 Maintenance of the Reduced State of DsbC by the Electron Transport Catalyst DsbD in the Inner Membrane | 189 | ||
3.1.3.3 DsbG, a Structural Homolog of DsbC with Sulfenic Acid Reductase Activity | 193 | ||
3.1.3.4 The Cytochrome c Maturation Factor CcmG is a DsbD Substrate | 194 | ||
3.1.4 Coexistence of the Oxidative Disulfide Bond Formation and the Reductive Disulfide Isomerization Pathways | 195 | ||
3.1.5 The Dsb System as a Potential Target for New Antibacterial Drugs | 197 | ||
3.1.6 Concluding Remarks | 199 | ||
Acknowledgements | 199 | ||
References | 199 | ||
Chapter 3.2 - Disulfide Bond Formation in Mitochondria | 205 | ||
3.2.1 Introduction | 205 | ||
3.2.2 Protein Import into the IMS | 206 | ||
3.2.3 Mia40, an Import Receptor in the IMS | 207 | ||
3.2.3.1 Structural Elements That Are Present in All Mia40 Proteins | 208 | ||
3.2.3.2 Kingdom-specific Domains in Mia40 Proteins | 209 | ||
3.2.4 Erv1, a Sulfhydryl Oxidase in the IMS | 209 | ||
3.2.4.1 Structural Organization of the Yeast Erv1 | 210 | ||
3.2.4.2 Structural Organization of the Other Erv1 Homologs | 211 | ||
3.2.5 Protein Import and Folding by the Mitochondrial Disulfide Relay | 211 | ||
3.2.5.1 Translocation of IMS Proteins Through the TOM Complex | 212 | ||
3.2.5.2 Mia40 Promotes Protein Translocation into the IMS | 213 | ||
3.2.5.3 Mia40 Promotes Oxidative Protein Folding in the IMS | 214 | ||
3.2.6 Substrate Proteins of the Mitochondrial Disulfide Relay | 214 | ||
3.2.6.1 Proteins of the Twin Cx3C Family | 215 | ||
3.2.6.2 Proteins of the Twin Cx9C Family | 216 | ||
3.2.6.3 IMS Proteins with Other Cysteine Patterns | 216 | ||
3.2.6.3.1 Ccs1 | 216 | ||
3.2.6.3.2 Atp23 | 216 | ||
3.2.6.3.3 MICU1 | 217 | ||
3.2.6.4 Inner Membrane Proteins | 217 | ||
3.2.6.4.1 Tim17 and Tim22 | 217 | ||
3.2.7 Perspectives | 217 | ||
References | 218 | ||
Chapter 3.3 - Structural Insights into Disulfide Bond Formation and Protein Quality Control in the Mammalian Endoplasmic Reticulum | 224 | ||
3.3.1 Introduction | 224 | ||
3.3.2 U-shaped Overall Structures of PDI and ERp57 | 226 | ||
3.3.2.1 Redox-dependent Regulation of PDI Structure and Function | 226 | ||
3.3.2.2 Regulated and Targeted PDI Oxidation by ER Oxidoreductin-1 (Ero1) | 228 | ||
3.3.2.3 Possible Homodimerization of PDI | 228 | ||
3.3.2.4 Structure, Function and Mechanism of ERp57 | 229 | ||
3.3.2.5 Structure and Possible Functions of ERp27 | 229 | ||
3.3.3 Structures and Functions of ERp46 and P5 | 231 | ||
3.3.3.1 Peroxiredoxin-4 (Prx4) is an Alternative Oxidase of PDIs | 231 | ||
3.3.3.2 Overall Structure of ERp46, an Efficient Protein Disulfide Introducer | 231 | ||
3.3.3.3 Schematic Structure and Possible Functions of P5 | 232 | ||
3.3.3.4 Modes of Interaction Between Prx4 and P5/ERp46 | 233 | ||
3.3.4 Structure, Function and Molecular Dynamism of ERdj5 | 233 | ||
3.3.4.1 The Highly Dynamic ERdj5 Adopts Two Alternative Conformations | 233 | ||
3.3.4.2 Novel Function of ERdj5 as a Modulator of Ca2+ Concentration in the ER | 236 | ||
3.3.5 ERp44 is a pH-sensing ESP Chaperone | 236 | ||
3.3.5.1 ERp44 Serves as a Second Checkpoint in the Post-ER Pathway | 236 | ||
3.3.5.2 ERp44 Has a Unique Structure | 237 | ||
3.3.5.3 ERp44 Undergoes pH-dependent Regulation | 239 | ||
3.3.5.4 Mechanism of Client Recognition by ERp44 | 241 | ||
3.3.6 Concluding Remarks | 243 | ||
Acknowledgements | 243 | ||
References | 243 | ||
Chapter 3.4 - Mechanisms of Oxidative Protein Folding and Thiol-dependent Quality Control: Tales of Cysteines and Cystines | 249 | ||
3.4.1 Introduction | 249 | ||
3.4.2 A Brief History of Protein Quality Control in the Early Secretory Pathway | 250 | ||
3.4.3 Thiol-dependent Quality Control | 251 | ||
3.4.3.1 Mechanisms of TDQC | 251 | ||
3.4.3.2 TDQC: Looking for the Culprits | 252 | ||
3.4.3.3 ERp44 Mediates TDQC | 253 | ||
3.4.4 Two Sequential QC Steps in the Early Secretory Pathway (ESP) | 254 | ||
3.4.5 Atomic Structure of ERp44: a Clever Clover | 254 | ||
3.4.6 The Sophisticated Clientele of ERp44 | 256 | ||
3.4.6.1 IgM | 257 | ||
3.4.6.2 Adiponectin | 258 | ||
3.4.6.3 A Mechanism for Controlling the Localization of ER Enzymes | 259 | ||
3.4.6.4 ERp44 TDQC: from the Substrate Point of View | 260 | ||
3.4.7 A Multifunctional Protein with a Selected Clientele | 261 | ||
3.4.8 Intersections Between TDQC and pH | 261 | ||
3.4.9 Concluding Remarks | 262 | ||
Acknowledgements | 263 | ||
References | 263 | ||
Chapter 3.5 - Disulfide Bond Formation Downstream of the Endoplasmic Reticulum | 267 | ||
3.5.1 Introduction | 267 | ||
3.5.2 Disulfide Rearrangements in the Golgi Apparatus | 269 | ||
3.5.3 Dedicated Catalysts of Disulfide Bond Formation Downstream of the Endoplasmic Reticulum: the QSOX Family | 271 | ||
3.5.3.1 QSOX Is a Golgi-localized and Secreted Catalyst of Disulfide Bond Formation | 271 | ||
3.5.3.2 QSOX Mechanism and Activity | 272 | ||
3.5.3.3 Function of QSOX in Cells and Organisms | 274 | ||
3.5.4 Oxidation of Immunoglobulins Outside the ER | 276 | ||
3.5.5 Extracellular PDI-mediated Disulfide Exchange in Neutrophil Adhesion and Thrombus Formation | 278 | ||
3.5.6 Unpaired Cysteines in Extracellular Proteins | 279 | ||
3.5.7 Conclusions and Perspectives | 280 | ||
References | 281 | ||
Section IV - Oxidative Folding and Cellular/Organism Homeostasis | 285 | ||
Chapter 4.1 - How Microbes Cope with Oxidative Stress | 287 | ||
4.1.1 Reactive Oxygen Species – an Inevitable Consequence of Aerobic Life | 287 | ||
4.1.2 Oxidative Stress – an Effective Mammalian Host Defense Mechanism | 288 | ||
4.1.3 Cellular Effects of Antimicrobial Oxidants | 288 | ||
4.1.4 How Bacteria Cope with Antimicrobial Oxidants | 289 | ||
4.1.4.1 Transcriptional Changes in Response to ROS and RCS | 289 | ||
4.1.4.1.1 The HOCl–N-Chlorotaurine Response System RclR | 290 | ||
4.1.4.1.2 The HOCl–Methylglyoxal Response System NemR | 291 | ||
4.1.4.1.3 The HOCl Response System HypT | 292 | ||
4.1.4.1.4 Other Bacterial Redox-sensitive Transcriptional Regulators | 292 | ||
4.1.4.2 Metabolic Changes Upon ROS–Antimicrobial Oxidant Insults | 293 | ||
4.1.4.3 ROS-mediated Activation of ATP-independent Molecular Chaperones | 293 | ||
4.1.4.3.1 Hsp33 – Activation by Reversible Disulfide Bond Formation | 294 | ||
4.1.4.3.2 RidA – Chaperone Activation by Methionine Oxidation | 295 | ||
4.1.5 Inorganic Polyphosphate: a Potent Protector Against Oxidative Damage | 296 | ||
4.1.5.1 PolyP Functions as a Potent Chaperone | 297 | ||
4.1.5.2 PolyP Production During Oxidative Stress | 297 | ||
4.1.5.3 The Many Other Hats of PolyP and Their Potential Roles in Oxidative Stress Protection | 298 | ||
4.1.6 Oxidative Stress-protective Systems as Novel Drug Targets | 299 | ||
4.1.7 Concluding Remarks | 299 | ||
References | 300 | ||
Chapter 4.2 - Disulfide Bond Formation in the Endoplasmic Reticulum | 306 | ||
4.2.1 Introduction | 306 | ||
4.2.2 Mechanism of Disulfide Formation in the ER | 307 | ||
4.2.3 The Environment of the ER is Optimized for Protein Folding | 308 | ||
4.2.3.1 The ER is a Repository for Folding Enzymes | 308 | ||
4.2.3.2 ER Calcium Concentration and Relevance to Oxidative Folding | 309 | ||
4.2.3.3 Glutathione Redox Buffer | 309 | ||
4.2.4 PDI Enzymes: Catalysts of Oxidative Folding | 310 | ||
4.2.4.1 Structure and Function of PDI | 313 | ||
4.2.5 ER Oxidative and Reductive Pathways | 315 | ||
4.2.5.1 The Ero1 Pathway | 315 | ||
4.2.5.2 ER Peroxidases Utilise Hydrogen Peroxide to Promote Disulfide Formation | 318 | ||
4.2.5.3 PrxIV Oxidative Pathway | 319 | ||
4.2.5.4 Glutathione Peroxidase7/8 Pathway | 321 | ||
4.2.5.5 Vitamin K Epoxide Reductase (VKOR) Pathway | 323 | ||
4.2.5.6 The ER Reductive Pathway | 324 | ||
4.2.6 Disulfide Exchange Between the PDI Proteins | 325 | ||
4.2.7 Conclusion | 327 | ||
Acknowledgements | 327 | ||
References | 328 | ||
Chapter 4.3 - Redox Regulation of Hsp70 Chaperone Function in the Endoplasmic Reticulum | 334 | ||
4.3.1 Introduction | 334 | ||
4.3.2 The ER-localized Hsp70 Chaperone System | 336 | ||
4.3.2.1 BiP: an Hsp70 Molecular Chaperone | 336 | ||
4.3.2.2 BiP Co-chaperones: J Proteins | 338 | ||
4.3.2.3 BiP Co-chaperones: Nucleotide Exchange Factors (NEFs) | 340 | ||
4.3.3 Modulating BiP Activity Under Oxidative Stress | 342 | ||
4.3.3.1 Formation of a BiP Cysteine Adduct | 342 | ||
4.3.3.2 Alterations to BiP Activities Upon Oxidation | 345 | ||
4.3.3.3 Reduction of Oxidized BiP | 346 | ||
4.3.4 Conclusions and Open Questions | 348 | ||
References | 349 | ||
Chapter 4.4 - Thioredoxin and Cellular Redox Systems: Beyond Protein Disulfide Bond Reduction | 355 | ||
4.4.1 Introduction | 355 | ||
4.4.1.1 Trx Superfamily Proteins | 356 | ||
4.4.1.2 The Thioredoxin System | 357 | ||
4.4.1.3 The Glutaredoxin System | 360 | ||
4.4.2 Functions of Trx and Grx Systems | 361 | ||
4.4.2.1 Protein Disulfide Isomerization | 361 | ||
4.4.2.2 Chaperone Activity | 363 | ||
4.4.2.3 Antioxidant System | 363 | ||
4.4.2.4 Electron Donors for RNR | 364 | ||
4.4.2.5 Iron–Sulfur Clusters | 365 | ||
4.4.3 Regulating Redox Signaling | 367 | ||
4.4.3.1 The Concept of Redox Signaling | 367 | ||
4.4.3.1.1 Sulfenic Acid and Sulfinic Acid | 367 | ||
4.4.3.1.2 Disulfide Bonds | 368 | ||
4.4.3.1.3 S-Glutathionylation | 368 | ||
4.4.3.1.4 S-Nitrosylation | 369 | ||
4.4.3.2 Redox Signaling Mediated by Trx Fold Proteins | 369 | ||
4.4.4 Concluding Remarks | 371 | ||
References | 372 | ||
Section V - Engineering Covalent Linkages in Peptides and Proteins | 379 | ||
Chapter 5.1 - Stabilization of Peptides and Proteins by Engineered Disulfide Bonds | 381 | ||
5.1.1 Protein Disulfide Bonds | 381 | ||
5.1.2 Considerations for Stability | 382 | ||
5.1.3 Computational Techniques and Tools | 384 | ||
5.1.3.1 Structure-based Design Tools | 385 | ||
5.1.3.1.1 PROTEUS | 385 | ||
5.1.3.1.2 SSBOND | 386 | ||
5.1.3.1.3 MODIP (Modeling of Disulfide Bridges in Proteins) | 386 | ||
5.1.3.1.4 DbD (Disulfide by Design) | 386 | ||
5.1.3.1.5 BridgeD | 387 | ||
5.1.3.1.6 GeoFold (Geometric Unfolding) | 387 | ||
5.1.3.2 Neural Network-based Design Tools | 387 | ||
5.1.3.2.1 DiANNA (Diamino Acid Neural Network Application) | 388 | ||
5.1.3.2.2 DISULFIND | 388 | ||
5.1.3.2.3 Dinosolve (Disulfide Bond Solve) | 388 | ||
5.1.3.3 Protein Engineering Suites | 389 | ||
5.1.3.3.1 Molecular Dynamics | 389 | ||
5.1.3.3.2 PIC (Protein Interaction Calculator) | 389 | ||
5.1.3.3.3 BioLuminate | 390 | ||
5.1.3.3.4 iRDP (In silico Rational Design of Proteins) | 390 | ||
5.1.3.3.5 MAESTRO | 390 | ||
5.1.3.4 Validation of Engineered Disulfides | 390 | ||
5.1.3.5 Tool and Suite Websites | 391 | ||
5.1.4 Applications of Engineered Disulfide Bonds | 391 | ||
5.1.4.1 Stabilization | 391 | ||
5.1.4.1.1 β-Glucanase | 392 | ||
5.1.4.1.2 Non-canonical Amino Acids Containing Long Side-chain Thiols | 392 | ||
5.1.4.1.3 α-Type Carbonic Anhydrase | 392 | ||
5.1.4.2 Functional and Structural Characterization, Refinement, and Design | 393 | ||
5.1.4.2.1 Insulin-degrading Enzyme (IDE) and Amyloid β-Protein | 393 | ||
5.1.4.2.2 Real-time Visualization of Perforin Nanopore Assembly | 393 | ||
5.1.4.2.3 Monomeric Streptavidin and Biotinylated Peptide Tag | 394 | ||
5.1.4.2.4 Diabody Engineering | 394 | ||
5.1.4.3 Crystallography and Structure | 394 | ||
5.1.4.4 Vaccines, Immunology and Drugs | 395 | ||
5.1.5 Conclusion | 396 | ||
References | 396 | ||
Chapter 5.2 - Genetic Code Expansion Approaches to Introduce Artificial Covalent Bonds into Proteins In Vivo | 399 | ||
5.2.1 Expanding the Genetic Code | 399 | ||
5.2.1.1 Site-specific Incorporation of Unnatural Amino Acids into Proteins | 399 | ||
5.2.1.2 Orthogonal tRNA Synthetase–tRNA Pairs | 400 | ||
5.2.1.3 Applications of Unnatural Amino Acids | 402 | ||
5.2.2 Bioorthogonal Reactions for Covalently Modifying Proteins | 404 | ||
5.2.2.1 Inverse Electron Demand Diels–Alder Cycloadditions for Fluorescently Labeling Proteins In vivo | 405 | ||
5.2.2.2 Inverse Electron Demand Diels–Alder Cycloadditions for Controlling Enzyme Activity in Living Cells | 407 | ||
5.2.3 Proximity-triggered Crosslinking | 408 | ||
5.2.3.1 Unnatural Amino Acids for Proximity-triggered Crosslinking | 409 | ||
5.2.3.2 Intramolecular and In vitro Intermolecular Proximity-triggered Protein Crosslinking | 410 | ||
5.2.3.3 In vivo Intermolecular Crosslinking for Covalent Stabilization of Low-affinity Protein Complexes | 414 | ||
5.2.4 Conclusion | 416 | ||
References | 417 | ||
Subject Index | 421 |