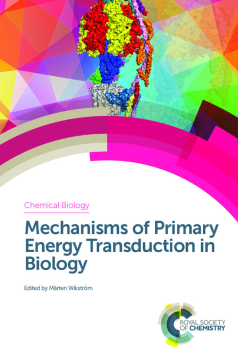
Additional Information
Book Details
Abstract
This book describes the events of primary energy transduction in life processes. Life as we know it depends on pumping protons across membranes. New tools to study the protein complexes involved has led to recent intensified progress in the field.
Primary Energy Transduction in Biology focusses on recent structural results and new biophysical insights. These have been made possible by recent advances in high-resolution protein structures, in physical techniques to study reactions in real time, and in computational methods to study and refine both structures and their dynamics. Written and edited by leading experts, chapters discuss the latest key questions in cell respiration, photosynthesis, bioenergetics, proton transfer, electron transfer and membrane transport.
Biochemists, biophysicists and chemical biologists will find this book an essential resource for a complete understanding of the molecular machines of bioenergetics.
Mårten Wikström received his MD, Ph.D. at the University of Helsinki in 1971, after which he spent a year as a postdoctoral researcher at the University of Amsterdam with Prof. E. C. Slater. In 1975−1976 he was visiting associate professor at the University of Pennsylvania with Prof. Britton Chance. He worked as an assistant professor at the University of Helsinki until 1983, when he was appointed to a personal Chair in medical chemistry (changed to physical biochemistry in 2002). In the period 1996−2006 he was Research Professor of the Academy of Finland. From 1998 to 2013 he was Research Director of the Structural Biology and Biophysics Program of the Institute of Biotechnology. He retired in 2013 but continues as Emeritus Professor. He is a recipient of the Anniversary Prize of the Federation of European Biochemical Societies (FEBS) in 1977, the Scandinavian Anders Jahre Prize in medicine in 1984 and 1996, and the David Keilin Prize and Medal (British Biochemical Society) in 1997, and he gave the Peter Mitchell Medal Lecture in 2000. He is an elected member of Societas Scientiarum Fennica (1982), the European Molecular Biology Organization (1985), The Royal Swedish Academy of Sciences (chemistry, 1992), and Academia Europaea (2010). His research interests are in molecular bioenergetics, membrane proteins, electron transfer, proton translocation, and mitochondrial diseases.
Table of Contents
Section Title | Page | Action | Price |
---|---|---|---|
Cover | Cover | ||
Preface | v | ||
Contents | vii | ||
Chapter 1 Making Maquette Models of Bioenergetic Structures | 1 | ||
1.1 Unravelling Protein Complexity | 1 | ||
1.2 Robust, Modular, Helix-bundle Scaffold for Electron-transfer Architecture | 2 | ||
1.3 Securing Cofactors with Histidines | 5 | ||
1.3.1 Hemes | 5 | ||
1.3.2 Non-iron Tetrapyrroles | 7 | ||
1.4 Securing Cofactors with Cysteines | 7 | ||
1.4.1 Heme C and Bilins | 7 | ||
1.4.2 Iron-Sulfur Clusters | 8 | ||
1.5 Securing Metals with Cys, His or Carboxylates | 8 | ||
1.6 Redox Active Amino Acids | 8 | ||
1.7 Practical Design Rules for Intraprotein Electron Tunnelling | 9 | ||
1.8 Bioenergetic Function Examples | 11 | ||
1.8.1 Ligand Binding and Transport | 11 | ||
1.8.2 Excitation Energy Transfer | 12 | ||
1.8.3 Interprotein Electron Transfer | 13 | ||
1.8.4 Intraprotein Electron Transfer | 14 | ||
1.9 Photosynthetic Charge Separation Engineering | 15 | ||
1.9.1 Photochemical Dyad and Triad Dynamics | 15 | ||
1.9.2 Photochemical Tyrosine Oxidation | 16 | ||
1.9.3 Photochemical Metal Cluster Oxidation | 17 | ||
1.10 Conclusion | 18 | ||
Acknowledgments | 19 | ||
References | 19 | ||
Chapter 2 Structure of Respiratory Complex I: ‘‘Minimal\" Bacterial and ‘‘De luxe\" Mammalian Versions | 25 | ||
2.1 Introduction | 25 | ||
2.2 Overview of the Bacterial Enzyme Structure | 30 | ||
2.3 Electron Transfer Pathway | 32 | ||
2.3.1 NADH/FMN Binding Site | 32 | ||
2.3.2 Fe-S Clusters | 32 | ||
2.3.3 Quinone Binding Site | 35 | ||
2.3.4 Cluster N1a and ROS Production | 36 | ||
2.4 Proton-translocating Channels | 37 | ||
2.5 Additional Subunits in Bacterial Enzyme | 39 | ||
2.6 Coupling Between Electron Transfer and Proton Translocation | 40 | ||
2.7 Recent Structural Studies on Mammalian Complex I | 41 | ||
2.8 Structure of the Ovine Mitochondrial Complex I | 43 | ||
2.8.1 Core Subunits | 43 | ||
2.8.2 Supernumerary Subunits | 45 | ||
2.8.3 Cofactors in Supernumerary Subunits | 47 | ||
2.9 Mitochondrial Complex I Mechanism: Similar to Bacterial but with Extra ‘‘Stabilisers\" and ‘‘Sensors | 50 | ||
References | 52 | ||
Chapter 3 Structure and Function of Respiratory Complex I | 60 | ||
3.1 Introduction | 60 | ||
3.2 Overall Structure of Complex I | 63 | ||
3.3 Accessory Subunits | 64 | ||
3.4 Central Subunits of the Membrane Arm | 65 | ||
3.5 Proton Translocation Pathways and the Central Hydrophilic Axis | 67 | ||
3.6 Peripheral Arm and Ubiquinone Binding Site | 69 | ||
3.7 Structural Basis of the A/D transition | 71 | ||
3.8 Catalytic Mechanism of Redox Linked Proton Translocation | 73 | ||
Acknowledgments | 76 | ||
References | 76 | ||
Chapter 4 Multi-scale Molecular Simulations on Respiratory Complex I | 81 | ||
4.1 Introduction to Structure and Function of Complex I | 81 | ||
4.2 Computational Models and Methods | 83 | ||
4.2.1 Classical Molecular Dynamics Simulations | 83 | ||
4.2.2 Free-energies and Electrostatic Poisson-Boltzmann Calculations | 86 | ||
4.2.3 Quantum Chemical Density Functional Theory Models | 87 | ||
4.2.4 Hybrid Quantum Mechanics/Classical Mechanics Models | 89 | ||
4.3 Dynamics of Electron Transfer | 90 | ||
4.4 Mechanism of Quinone Reduction | 92 | ||
4.5 Redox-linked Conformational Changes in the Membrane Domain | 94 | ||
4.6 Function of the Proton Pump | 95 | ||
4.7 Putative Model for Redox-driven Proton-pumping | 97 | ||
4.8 Conclusions | 98 | ||
Abbreviations | 98 | ||
Acknowledgments | 99 | ||
References | 99 | ||
Chapter 5 Coupling Hydride Transfer to Proton Pumping: the Swiveling Mechanism of Transhydrogenase | 104 | ||
5.1 Introduction | 104 | ||
5.2 Location and Physiological Roles of Transhydrogenase | 105 | ||
5.3 Significance of the Transhydrogenase to Human Health | 107 | ||
5.4 Domains, Subunits and Sequence Conservation | 108 | ||
5.4.1 Single-subunit Transhydrogenase, Variant 1 | 109 | ||
5.4.2 Single-subunit Transhydrogenase, Variant 2 | 109 | ||
5.4.3 Two-subunit Transhydrogenase | 109 | ||
5.4.4 Three-subunit Transhydrogenase | 111 | ||
5.5 Steady State Assays of the Transhydrogenase | 111 | ||
5.6 Structures of the Isolated Domains: Divide and Conquer | 113 | ||
5.6.1 Domain I | 113 | ||
5.6.2 Domain III | 116 | ||
5.6.3 Heterotrimer, (dI)2(dIII) | 117 | ||
5.6.4 The Membrane Domain (dII) | 119 | ||
5.7 Structure of the Holo-enzyme | 123 | ||
5.7.1 Two Orientations of Domain III | 123 | ||
5.7.2 Asymmetry of the Two Protomers of Domain II | 125 | ||
5.7.3 Proton Translocation and the Face-down Orientation of dIII | 126 | ||
5.8 Key Biochemical Observations | 126 | ||
5.8.1 Cooperativity Between the Two Protomers | 126 | ||
5.8.2 Direct Hydride Transfer Across the dI/dIII Interface | 126 | ||
5.8.3 Hydride Transfer Between Enzyme-bound \r\nDinucleotides Favors the Formation of NADPH | 127 | ||
5.9 Insights from Binding Studies | 129 | ||
5.9.1 Binding of NAD(H) to Domain I is Not Influenced by the Presence or Absence of the Membrane Domain | 129 | ||
5.9.2 Negative Cooperativity Prevents the Formation of ‘‘Dead End\" States of the Enzyme | 129 | ||
5.9.3 The Occluded State of dIII Has a Very High Apparent Affinity for NADP(H) | 129 | ||
5.10 Insights from Mutagenesis Studies of the Membrane Domain | 130 | ||
5.10.1 Mutations in the Middle of the Membrane Domain (dII) Influence Enzyme Activity, Proton Translocation and the Binding of NADP(H) | 130 | ||
5.10.2 Mutations in the Hinge Region (Ec-βK261 to Ec-βR265) and Ec-βD213 Inhibit Transhydrogenase Activity | 131 | ||
5.11 Working Model for Coupling Hydride Transfer and Proton Translocation Across the Membrane | 132 | ||
5.11.1 The Main Features of the Model are Briefly Summarized | 132 | ||
References | 134 | ||
Chapter 6 The Na+-Translocating NADH: Ubiquinone Oxidoreductase (Na+-NQR) | 140 | ||
6.1 Introduction | 140 | ||
6.2 The Electron Pathway | 141 | ||
6.3 A Possible Sixth Cofactor | 146 | ||
6.4 Defining Na+ Translocation Through Acidic Residues | 147 | ||
6.5 Defining Na+ Binding Sites | 149 | ||
6.6 Discovery of the First Na+ Binding Site | 149 | ||
6.7 Localization of the First Na+ Binding Site in the Crystal Structure | 151 | ||
6.8 Location of a Second Na+ Binding Site | 154 | ||
6.9 Perspectives | 156 | ||
Acknowledgments | 156 | ||
References | 156 | ||
Chapter 7 The bc1 Complex: A Physicochemical Retrospective and an Atomistic Prospective | 161 | ||
7.1 Introduction | 161 | ||
7.2 Current Paradigm: The Monomeric Q-cycle Mechanism of the bc1 Complex | 162 | ||
7.3 Control and Gating | 164 | ||
7.4 The Marcus-Brønsted Mechanism for First Electron Transfer of the Q-cycle | 167 | ||
7.5 The Dimer Interface | 169 | ||
7.5.1 The ISP Subunit and Its Clamp | 169 | ||
7.5.2 Electron Transfer Between the bL Hemes | 169 | ||
7.5.3 Coulombic Interactions Across the Interface | 170 | ||
7.5.4 The ‘void' Between the Monomers Seen in Crystallographic Structures | 171 | ||
7.6 Recent Developments | 172 | ||
7.6.1 A New Gating Mechanism in the First Electron Transfer | 172 | ||
7.6.2 Dissecting the Second Electron Transfer | 173 | ||
7.6.3 The Semiquinone Intermediate of the Bifurcated Reaction | 173 | ||
7.6.4 A New Intermediate SQo Complex | 173 | ||
7.6.5 How Is the Electron Pair in the SQo•.ISPH• Complex Entangled? | 176 | ||
7.7 Proton Release Associated with Qo-site Turnover | 177 | ||
7.8 A Model of the Rb. sphaeroides bc1 Complex for MD Simulation in a Native Membrane | 180 | ||
7.8.1 The Qo-site | 183 | ||
7.8.2 The Qi-site | 186 | ||
Acknowledgments | 187 | ||
References | 187 | ||
Chapter 8 Advances in Understanding Mechanism and Physiology of Cytochromes bc | 192 | ||
8.1 Introduction | 192 | ||
8.1.1 Overview of Cytochrome bc Complexes | 192 | ||
8.1.2 Structure of Cytochrome bc1 | 193 | ||
8.1.3 Catalytic Cycle | 195 | ||
8.1.4 Purpose of This Review | 196 | ||
8.2 New Insights into Operation of the Qo Catalytic Site | 196 | ||
8.2.1 Electron and Proton Routes at the Qo Site | 196 | ||
8.2.2 Semiquinone at the Qo Site and Its Involvement in Superoxide Generation | 198 | ||
8.2.3 Testing the ‘‘Semireverse\" Model of ROS Production Using Mitochondrial Mutations | 202 | ||
8.2.4 Physiological Aspects of Superoxide Generation at the Qo Site | 202 | ||
8.2.5 Possible Role of Metastable State of Semiquinone: Rieske Cluster in Protection Against ROS | 203 | ||
8.3 New Insights into Proton Transfer at the Qi Site | 205 | ||
8.4 Cytochrome bc1 as a Functional Dimer | 208 | ||
8.5 Concluding Remarks | 208 | ||
Acknowledgments | 209 | ||
References | 209 | ||
Chapter 9 Life and Death of Cytochrome c Oxidase: Influence of Subunit III on the D pathway, Proton Backflow and Suicide Inactivation | 215 | ||
9.1 Introduction | 215 | ||
9.2 A Short Primer on Cytochrome c Oxidase | 216 | ||
9.3 Subunit III Structure | 218 | ||
9.3.1 Subunit III Binds Lipid in an Internal Cleft | 219 | ||
9.3.2 Lipids of Subunit III Play a Key Role in the Subunit I-III Interaction | 222 | ||
9.3.3 Connecting Subunit III to the Active Site Region of Subunit I | 223 | ||
9.3.4 Relationship Between Subunit III and the D Pathway | 224 | ||
9.3.5 Removal of Subunit III | 224 | ||
9.4 Subunit III and the D Pathway | 225 | ||
9.4.1 Normal D Pathway Structure and Function | 226 | ||
9.4.2 Rapid Kinetic Analysis Reveals D Path Function | 226 | ||
9.4.3 The pH Dependence of the D Pathway is Strongly Shifted in the Absence of Subunit III. Why? | 228 | ||
9.4.4 Steady-state Proton Uptake in the Absence of Subunit III Can Report the pKa and the Rate of D Pathway Proton Uptake | 229 | ||
9.5 Subunit III & Proton Pumping | 231 | ||
9.6 Subunit III & Suicide Inactivation | 231 | ||
9.7 Mechanism of Suicide Inactivation | 232 | ||
9.8 Structural Influence of Subunit III Helps Prevent SI, and Involves its Bound Lipids | 236 | ||
9.9 The Histidines Near the Entrance of the D Pathway: Proton Antenna or Not? | 238 | ||
9.10 Proton Backflow and SI | 240 | ||
9.10.1 Identification of Proton Backflow | 240 | ||
9.10.2 The Mutant D132A as a System to Study Proton Backflow | 241 | ||
9.10.3 Proton Backflow versus Proton Exit | 241 | ||
9.10.4 Functional Significance of Proton Backflow In vivo | 242 | ||
9.10.5 Subunit III Appears to be Required for a Functional Proton Backflow Pathway | 242 | ||
9.11 Subunit III and O2 Delivery | 243 | ||
9.12 Summary | 243 | ||
References | 244 | ||
Chapter 10 Computational Means of Assessing Proton Pumping in Cytochrome c Oxidase (Complex IV) | 249 | ||
10.1 Introduction | 249 | ||
10.2 Catalytic Cycle | 252 | ||
10.3 Proton Pumping Mechanism | 254 | ||
10.4 Simulating PT in Biomolecular Systems | 255 | ||
10.5 Hydration and Proton Transport | 256 | ||
10.6 Transport of the Pumped Proton | 259 | ||
10.7 Transport of the Chemical Proton | 261 | ||
10.8 Proton Transport through the D-Channel | 264 | ||
10.9 Conclusions | 267 | ||
References | 268 | ||
Chapter 11 Water Oxidation by PSII: A Quantum Chemical Approach | 273 | ||
11.1 Introduction | 273 | ||
11.2 Methods and Models | 277 | ||
11.3 Discussion | 278 | ||
11.3.1 S-state Structures | 279 | ||
11.3.2 O-O Bond Formation | 281 | ||
11.3.3 Full Energy Diagram | 283 | ||
11.3.4 The Effects of Tyrz | 286 | ||
11.3.5 The Importance of Calcium | 288 | ||
11.3.6 Water Insertion in the S2 to S3 Transition | 290 | ||
11.4 Summary | 291 | ||
Acknowledgments | 292 | ||
References | 292 | ||
Chapter 12 Respiratory Supercomplexes in Mitochondria | 296 | ||
12.1 Introduction | 296 | ||
12.1.1 The Respiratory Chain of Mitochondria | 296 | ||
12.1.2 Organization of the Respiratory Chain: Historical Outline | 298 | ||
12.2 Distribution and Composition of Respiratory Supercomplexes | 300 | ||
12.2.1 Distribution in Different Organisms | 300 | ||
12.2.2 Composition of Respiratory Supercomplexes | 300 | ||
12.3 Supercomplex Association Provides a Kinetic Advantage | 303 | ||
12.3.1 Structural Evidence | 305 | ||
12.3.2 Evidence for Channelling in the Coenzyme Q Region | 310 | ||
12.3.3 Electron Transfer Through Cytochrome c | 318 | ||
12.4 Supercomplexes and Reactive Oxygen Species | 321 | ||
12.5 Physiological and Pathological Implications | 324 | ||
12.5.1 Supercomplexes and Regulation of Metabolic Fluxes | 324 | ||
12.5.2 Supercomplexes and ROS Signalling | 326 | ||
12.5.3 Supercomplexes in Pathology and Aging | 327 | ||
References | 329 | ||
Chapter 13 Structure, Mechanism and Regulation of ATP Synthases | 338 | ||
13.1 Introduction | 338 | ||
13.2 Structure of ATP Synthases | 339 | ||
13.2.1 Subunit Compositions | 339 | ||
13.2.2 High Resolution Structure | 342 | ||
13.3 Catalytic Mechanism of ATP Synthases | 344 | ||
13.3.1 ATP Hydrolysis | 344 | ||
13.3.2 Structural Description of the Rotary Catalytic Cycle | 346 | ||
13.3.3 Generation of Rotation in the Membrane Domain | 348 | ||
13.3.4 Role of Cardiolipin | 351 | ||
13.3.5 Bioenergetic Cost of Making an ATP Molecule | 351 | ||
13.4 Regulatory Mechanisms | 353 | ||
13.4.1 Mitochondrial ATP Synthases | 353 | ||
13.4.2 Bacterial ATP Synthases | 356 | ||
13.5 Perspectives | 359 | ||
13.5.1 Determination of Structures of ATP Synthases | 359 | ||
13.5.2 The Catalytic Cycle and Rotary Mechanism | 361 | ||
13.5.3 The Peripheral Stalk | 362 | ||
13.5.4 The Mitochondrial Inhibitor Protein | 364 | ||
13.5.5 ATP Synthase as a Drug Target | 364 | ||
13.5.6 The Permeability Transition Pore | 365 | ||
Note Added in Proof | 366 | ||
Acknowledgments | 366 | ||
References | 366 | ||
Subject Index | 374 |