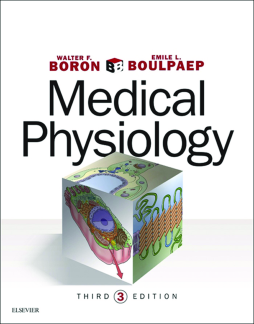
Additional Information
Book Details
Abstract
For a comprehensive understanding of human physiology — from molecules to systems —turn to the latest edition of Medical Physiology. This updated textbook is known for its unparalleled depth of information, equipping students with a solid foundation for a future in medicine and healthcare, and providing clinical and research professionals with a reliable go-to reference. Complex concepts are presented in a clear, concise, and logically organized format to further facilitate understanding and retention.
- Clear, didactic illustrations visually present processes in a clear, concise manner that is easy to understand.
- Intuitive organization and consistent writing style facilitates navigation and comprehension.
- Takes a strong molecular and cellular approach that relates these concepts to human physiology and disease.
- An increased number of clinical correlations provides a better understanding of the practical applications of physiology in medicine.
- Highlights new breakthroughs in molecular and cellular processes, such as the role of epigenetics, necroptosis, and ion channels in physiologic processes, to give insights into human development, growth, and disease.
- Several new authors offer fresh perspectives in many key sections of the text, and meticulous editing makes this multi-authored resource read with one unified voice.
- Includes electronic access to 10 animations and copious companion notes prepared by the Editors.
Table of Contents
Section Title | Page | Action | Price |
---|---|---|---|
Front Cover | cover | ||
Inside Front Cover | ifc1 | ||
IFC_International edition | IFC1 | ||
Medical Physiology | i | ||
Copyright Page | iv | ||
Contributors | v | ||
Video Table of Contents | vi | ||
Preface to the Third Edition | vii | ||
The eBook | vii | ||
Acknowledgments | vii | ||
Preface to the First Edition | ix | ||
Target Audience | ix | ||
Content of the Textbook | ix | ||
Emphasis of the Textbook | ix | ||
Creating the Textbook | ix | ||
Special Features | x | ||
Acknowledgments | x | ||
Table Of Contents | xi | ||
I Introduction | 1 | ||
1 Foundations of Physiology | 2 | ||
What is physiology? | 2 | ||
Physiological genomics is the link between the organ and the gene | 2 | ||
Cells live in a highly protected milieu intérieur | 3 | ||
Homeostatic mechanisms—operating through sophisticated feedback control mechanisms— are responsible for maintaining the constancy of the milieu intérieur | 4 | ||
Medicine is the study of “physiology gone awry” | 4 | ||
References | 5 | ||
References | 5.e1 | ||
II Physiology of Cells and Molecules | 7 | ||
2 Functional Organization of the Cell | 8 | ||
Structure of Biological Membranes | 8 | ||
The surface of the cell is defined by a membrane | 8 | ||
The cell membrane is composed primarily of phospholipids | 8 | ||
Phospholipids form complex structures in aqueous solution | 9 | ||
The diffusion of individual lipids within a leaflet of a bilayer is determined by the chemical makeup of its constituents | 10 | ||
Phospholipid bilayer membranes are impermeable to charged molecules | 11 | ||
The plasma membrane is a bilayer | 12 | ||
Membrane proteins can be integrally or peripherally associated with the plasma membrane | 13 | ||
The membrane-spanning portions of transmembrane proteins are usually hydrophobic α helices | 13 | ||
Some membrane proteins are mobile in the plane of the bilayer | 15 | ||
Function of Membrane Proteins | 16 | ||
Integral membrane proteins can serve as receptors | 16 | ||
Integral membrane proteins can serve as adhesion molecules | 17 | ||
Integral membrane proteins can carry out the transmembrane movement of water-soluble substances | 17 | ||
Integral membrane proteins can also be enzymes | 18 | ||
Integral membrane proteins can participate in intracellular signaling | 18 | ||
Peripheral membrane proteins participate in intracellular signaling and can form a submembranous cytoskeleton | 18 | ||
Cellular Organelles and the Cytoskeleton | 20 | ||
The cell is composed of discrete organelles that subserve distinct functions | 20 | ||
The nucleus stores, replicates, and reads the cell’s genetic information | 21 | ||
Lysosomes digest material derived from the interior and exterior of the cell | 22 | ||
The mitochondrion is the site of oxidative energy production | 22 | ||
The cytoplasm is not amorphous but is organized by the cytoskeleton | 22 | ||
Intermediate filaments provide cells with structural support | 23 | ||
Microtubules provide structural support and provide the basis for several types of subcellular motility | 23 | ||
Thin filaments (actin) and thick filaments (myosin) are present in almost every cell type | 25 | ||
Synthesis and Recycling of Membrane Proteins | 28 | ||
Secretory and membrane proteins are synthesized in association with the rough ER | 28 | ||
Simultaneous protein synthesis and translocation through the rough ER membrane requires machinery for signal recognition and protein translocation | 28 | ||
Proper insertion of membrane proteins requires start- and stop-transfer sequences | 30 | ||
Newly synthesized secretory and membrane proteins undergo post-translational modification and folding in the lumen of the rough ER | 32 | ||
Secretory and membrane proteins follow the secretory pathway through the cell | 34 | ||
Carrier vesicles control the traffic between the organelles of the secretory pathway | 35 | ||
Specialized protein complexes, such as clathrin and coatamers, mediate the formation and fusion of vesicles in the secretory pathway | 35 | ||
Vesicle Formation in the Secretory Pathway | 35 | ||
Vesicle Fusion in the Secretory Pathway | 37 | ||
Newly synthesized secretory and membrane proteins are processed during their passage through the secretory pathway | 37 | ||
Newly synthesized proteins are sorted in the trans-Golgi network | 39 | ||
A mannose-6-phosphate recognition marker is required to target newly synthesized hydrolytic enzymes to lysosomes | 40 | ||
Cells internalize extracellular material and plasma membrane through the process of endocytosis | 40 | ||
Receptor-mediated endocytosis is responsible for internalizing specific proteins | 42 | ||
Endocytosed proteins can be targeted to lysosomes or recycled to the cell surface | 42 | ||
Certain molecules are internalized through an alternative process that involves caveolae | 42 | ||
Specialized Cell Types | 43 | ||
Epithelial cells form a barrier between the internal and external milieu | 43 | ||
Tight Junctions | 43 | ||
Adhering Junctions | 44 | ||
Gap Junctions | 45 | ||
Desmosomes | 45 | ||
Epithelial cells are polarized | 45 | ||
References | 46 | ||
References | 46.e1 | ||
Books and Reviews | 46.e1 | ||
Journal Articles | 46.e1 | ||
3 Signal Transduction | 47 | ||
Mechanisms of Cellular Communication | 47 | ||
Cells can communicate with one another via chemical signals | 47 | ||
Soluble chemical signals interact with target cells via binding to surface or intracellular receptors | 47 | ||
Cells can also communicate by direct interactions—juxtacrine signaling | 50 | ||
Gap Junctions | 50 | ||
Adhering and Tight Junctions | 50 | ||
Membrane-Associated Ligands | 50 | ||
Ligands in the Extracellular Matrix | 50 | ||
Second-messenger systems amplify signals and integrate responses among cell types | 50 | ||
Receptors That are Ion Channels | 51 | ||
Ligand-gated ion channels transduce a chemical signal into an electrical signal | 51 | ||
Receptors Coupled to G Proteins | 51 | ||
General Properties of G Proteins | 52 | ||
G proteins are heterotrimers that exist in many combinations of different α, β, and γ subunits | 52 | ||
G-protein activation follows a cycle | 53 | ||
Activated α subunits couple to a variety of downstream effectors, including enzymes and ion channels | 53 | ||
βγ subunits can activate downstream effectors | 56 | ||
Small GTP-binding proteins are involved in a vast number of cellular processes | 56 | ||
G-Protein Second Messengers: Cyclic Nucleotides | 56 | ||
cAMP usually exerts its effect by increasing the activity of protein kinase A | 56 | ||
Protein phosphatases reverse the action of kinases | 57 | ||
cGMP exerts its effect by stimulating a nonselective cation channel in the retina | 58 | ||
G-Protein Second Messengers: Products of Phosphoinositide Breakdown | 58 | ||
Many messengers bind to receptors that activate phosphoinositide breakdown | 58 | ||
IP3 liberates Ca2+ from intracellular stores | 60 | ||
Calcium activates calmodulin-dependent protein kinases | 60 | ||
DAGs and Ca2+ activate protein kinase C | 60 | ||
G-Protein Second Messengers: Arachidonic Acid Metabolites | 61 | ||
Phospholipase A2 is the primary enzyme responsible for releasing AA | 62 | ||
Cyclooxygenases, lipoxygenases, and epoxygenases mediate the formation of biologically active eicosanoids | 62 | ||
Prostaglandins, prostacyclins, and thromboxanes (cyclooxygenase products) are vasoactive, regulate platelet action, and modulate ion transport N3-16 | 64 | ||
The leukotrienes (5-lipoxygenase products) play a major role in inflammatory responses | 64 | ||
The HETEs and EETs (epoxygenase products) tend to enhance Ca2+ release from intracellular stores and to enhance cell proliferation | 65 | ||
Degradation of the eicosanoids terminates their activity | 65 | ||
Receptors That are Catalytic | 66 | ||
The receptor guanylyl cyclase transduces the activity of atrial natriuretic peptide, whereas a soluble guanylyl cyclase transduces the activity of nitric oxide | 66 | ||
Receptor (Membrane-Bound) Guanylyl Cyclase | 66 | ||
Soluble Guanylyl Cyclase | 66 | ||
Some catalytic receptors are serine/threonine kinases | 67 | ||
RTKs produce phosphotyrosine motifs recognized by SH2 and phosphotyrosine-binding domains of downstream effectors | 68 | ||
Creation of Phosphotyrosine Motifs | 68 | ||
Recognition of pY Motifs by SH2 and Phosphotyrosine-Binding Domains | 68 | ||
The MAPK Pathway | 68 | ||
The Phosphatidylinositol-3-Kinase Pathway | 69 | ||
Tyrosine kinase–associated receptors activate cytosolic tyrosine kinases such as Src and JAK | 70 | ||
Receptor tyrosine phosphatases are required for lymphocyte activation | 71 | ||
Nuclear Receptors | 71 | ||
Steroid and thyroid hormones enter the cell and bind to members of the nuclear receptor superfamily in the cytoplasm or nucleus | 71 | ||
Activated nuclear receptors bind to sequence elements in the regulatory region of responsive genes and either activate or repress DNA transcription | 72 | ||
References | 72 | ||
References | 72.e1 | ||
Books and Reviews | 72.e1 | ||
Journal Articles | 72.e1 | ||
4 Regulation of Gene Expression | 73 | ||
From Genes to Proteins | 73 | ||
Gene expression differs among tissues and—in any tissue—may vary in response to external stimuli | 73 | ||
Genetic information flows from DNA to proteins | 73 | ||
The gene consists of a transcription unit | 74 | ||
DNA is packaged into chromatin | 75 | ||
Gene expression may be regulated at multiple steps | 76 | ||
Transcription factors are proteins that regulate gene transcription | 78 | ||
The Promoter and Regulatory Elements | 78 | ||
The basal transcriptional machinery mediates gene transcription | 78 | ||
The promoter determines the initiation site and direction of transcription | 78 | ||
Positive and negative regulatory elements modulate gene transcription | 79 | ||
Locus control regions and insulator elements influence transcription within multigene chromosomal domains | 80 | ||
Transcription Factors | 81 | ||
DNA-binding transcription factors recognize specific DNA sequences | 81 | ||
Transcription factors that bind to DNA can be grouped into families based on tertiary structure | 82 | ||
Zinc Finger | 82 | ||
Basic Zipper | 83 | ||
Basic Helix-Loop-Helix | 83 | ||
Helix-Turn-Helix | 83 | ||
Coactivators and corepressors are transcription factors that do not bind to DNA | 83 | ||
Transcriptional activators stimulate transcription by three mechanisms | 84 | ||
Recruitment of the Basal Transcriptional Machinery | 84 | ||
Chromatin Remodeling | 84 | ||
Stimulation of Pol II | 85 | ||
Transcriptional activators act in combination | 85 | ||
Transcriptional repressors act by competition, quenching, or active repression | 85 | ||
The activity of transcription factors may be regulated by post-translational modifications | 86 | ||
Phosphorylation | 86 | ||
Site-Specific Proteolysis | 87 | ||
Other Post-Translational Modifications | 88 | ||
The expression of some transcription factors is tissue specific | 88 | ||
Regulation of Inducible Gene Expression by Signal-Transduction Pathways | 89 | ||
cAMP regulates transcription via the transcription factors CREB and CBP | 89 | ||
Receptor tyrosine kinases regulate transcription via a Ras-dependent cascade of protein kinases | 89 | ||
Tyrosine kinase–associated receptors can regulate transcription via JAK-STAT | 90 | ||
Nuclear receptors are transcription factors | 90 | ||
Modular Construction | 90 | ||
Dimerization | 90 | ||
Activation of Transcription | 90 | ||
Repression of Transcription | 92 | ||
Physiological stimuli can modulate transcription factors, which can coordinate complex cellular responses | 92 | ||
Epigenetic Regulation of Gene Expression | 94 | ||
Epigenetic regulation can result in long-term gene silencing | 94 | ||
Alterations in chromatin structure may mediate epigenetic regulation, stimulating or inhibiting gene transcription | 94 | ||
Histone methylation may stimulate or inhibit gene expression | 94 | ||
DNA methylation is associated with gene inactivation | 95 | ||
Post-Translational Regulation of Gene Expression | 96 | ||
Alternative splicing generates diversity from single genes | 96 | ||
Retained Intron | 97 | ||
Alternative 3′ Splice Sites | 97 | ||
Alternative 5′ Splice Sites | 97 | ||
Cassette Exons | 97 | ||
Mutually Exclusive Exons | 97 | ||
Alternative 5′ Ends | 98 | ||
Alternative 3′ Ends | 98 | ||
Regulatory elements in the 3′ untranslated region control mRNA stability | 98 | ||
MicroRNAs regulate mRNA abundance and translation | 99 | ||
References | 100 | ||
References | 101.e1 | ||
Books and Reviews | 101.e1 | ||
Journal Articles | 101.e1 | ||
Glossary | 100 | ||
5 Transport of Solutes and Water | 102 | ||
The Intracellular and Extracellular Fluids | 102 | ||
Total-body water is the sum of the ICF and ECF volumes | 102 | ||
Plasma Volume | 102 | ||
Interstitial Fluid | 102 | ||
Transcellular Fluid | 102 | ||
ICF is rich in K+, whereas ECF is rich in Na+ and Cl− | 102 | ||
Volume Occupied by Plasma Proteins | 103 | ||
Effect of Protein Charge | 104 | ||
All body fluids have approximately the same osmolality, and each fluid has equal numbers of positive and negative charges | 105 | ||
Osmolality | 105 | ||
Electroneutrality | 105 | ||
Solute Transport Across Cell Membranes | 105 | ||
In passive, noncoupled transport across a permeable membrane, a solute moves down its electrochemical gradient | 105 | ||
At equilibrium, the chemical and electrical potential energy differences across the membrane are equal but opposite | 106 | ||
(Vm − EX) is the net electrochemical driving force acting on an ion | 107 | ||
In simple diffusion, the flux of an uncharged substance through membrane lipid is directly proportional to its concentration difference | 108 | ||
Some substances cross the membrane passively through intrinsic membrane proteins that can form pores, channels, or carriers | 108 | ||
Water-filled pores can allow molecules, some as large as 45 kDa, to cross membranes passively | 109 | ||
Gated channels, which alternately open and close, allow ions to cross the membrane passively | 110 | ||
Na+ Channels | 111 | ||
K+ Channels | 111 | ||
Ca2+ Channels | 111 | ||
Proton Channels | 111 | ||
Anion Channels | 111 | ||
Some carriers facilitate the passive diffusion of small solutes such as glucose | 111 | ||
The physical structures of pores, channels, and carriers are quite similar | 115 | ||
The Na-K pump, the most important primary active transporter in animal cells, uses the energy of ATP to extrude Na+ and take up K | 115 | ||
Besides the Na-K pump, other P-type ATPases include the H-K and Ca pumps | 117 | ||
H-K Pump | 117 | ||
Ca Pumps | 118 | ||
Other Pumps | 118 | ||
The F-type and the V-type ATPases transport H | 118 | ||
F-type or FoF1 ATPases | 118 | ||
V-type H Pump | 118 | ||
ATP-binding cassette transporters can act as pumps, channels, or regulators | 119 | ||
ABCA Subfamily | 119 | ||
MDR Subfamily | 120 | ||
MRP/CFTR Subfamily | 120 | ||
Cotransporters, one class of secondary active transporters, are generally driven by the energy of the inwardly directed Na+ gradient | 120 | ||
Na/Glucose Cotransporter | 121 | ||
Na+-Driven Cotransporters for Organic Solutes | 122 | ||
Na/HCO3 Cotransporters | 122 | ||
Na+-Driven Cotransporters for Other Inorganic Anions | 122 | ||
Na/K/Cl Cotransporter | 122 | ||
Na/Cl Cotransporter | 123 | ||
K/Cl Cotransporter | 123 | ||
H+-Driven Cotransporters | 123 | ||
Exchangers, another class of secondary active transporters, exchange ions for one another | 123 | ||
Na-Ca Exchanger | 123 | ||
Na-H Exchanger | 124 | ||
Na+-Driven Cl-HCO3 Exchanger | 124 | ||
Cl-HCO3 Exchanger | 124 | ||
Other Anion Exchangers | 125 | ||
Regulation of Intracellular Ion Concentrations | 125 | ||
The Na-K pump keeps [Na+] inside the cell low and [K+] high | 125 | ||
The Ca pump and the Na-Ca exchanger keep intracellular [Ca2+] four orders of magnitude lower than extracellular [Ca2+] | 126 | ||
Ca Pump (SERCA) in Organelle Membranes | 126 | ||
Ca Pump (PMCA) on the Plasma Membrane | 126 | ||
Na-Ca Exchanger (NCX) on the Plasma Membrane | 126 | ||
In most cells, [Cl−] is modestly above equilibrium because Cl− uptake by the Cl-HCO3 exchanger and Na/K/Cl cotransporter balances passive Cl− efflux through channels | 127 | ||
The Na-H exchanger and Na+-driven transporters keep the intracellular pH and [] above their equilibrium values | 127 | ||
Water Transport and the Regulation of Cell Volume | 127 | ||
Water transport is driven by osmotic and hydrostatic pressure differences across membranes | 127 | ||
Because of the presence of impermeant, negatively charged proteins within the cell, Donnan forces will lead to cell swelling | 128 | ||
The Na-K pump maintains cell volume by doing osmotic work that counteracts the passive Donnan forces | 130 | ||
Cell volume changes trigger rapid changes in ion channels or transporters, returning volume toward normal | 130 | ||
Response to Cell Shrinkage | 131 | ||
Response to Cell Swelling | 131 | ||
Cells respond to long-term hyperosmolality by accumulating new intracellular organic solutes | 132 | ||
The gradient in tonicity—or effective osmolality—determines the osmotic flow of water across a cell membrane | 132 | ||
Water Exchange Across Cell Membranes | 132 | ||
Water Exchange Across the Capillary Wall | 133 | ||
Adding isotonic saline, pure water, or pure NaCl to the ECF will increase ECF volume but will have divergent effects on ICF volume and ECF osmolality | 134 | ||
Infusion of Isotonic Saline | 135 | ||
Infusion of “Solute-Free” Water | 135 | ||
Ingestion of Pure NaCl Salt | 135 | ||
Whole-body Na+ content determines ECF volume, whereas whole-body water content determines osmolality | 135 | ||
Transport of Solutes and Water Across Epithelia | 136 | ||
The epithelial cell generally has different electrochemical gradients across its apical and basolateral membranes | 136 | ||
Tight and leaky epithelia differ in the permeabilities of their tight junctions | 136 | ||
Epithelial cells can absorb or secrete different solutes by inserting specific channels or transporters at either the apical or basolateral membrane | 137 | ||
Na+ Absorption | 137 | ||
K+ Secretion | 138 | ||
Glucose Absorption | 138 | ||
Cl− Secretion | 139 | ||
Water transport across epithelia passively follows solute transport | 139 | ||
Absorption of a Hyperosmotic Fluid | 139 | ||
Absorption of an Isosmotic Fluid | 139 | ||
Absorption of a Hypo-osmotic Fluid | 139 | ||
Epithelia can regulate transport by controlling transport proteins, tight junctions, and the supply of the transported substances | 139 | ||
Increased Synthesis (or Degradation) of Transport Proteins | 139 | ||
Recruitment of Transport Proteins to the Cell Membrane | 140 | ||
Post-translational Modification of Pre-existing Transport Proteins | 140 | ||
Changes in the Paracellular Pathway | 140 | ||
Luminal Supply of Transported Species and Flow Rate | 140 | ||
References | 140 | ||
References | 140.e1 | ||
Books and Reviews | 140.e1 | ||
Journal Articles | 140.e2 | ||
6 Electrophysiology of the Cell Membrane | 141 | ||
Ionic Basis of Membrane Potentials | 141 | ||
Principles of electrostatics explain why aqueous pores formed by channel proteins are needed for ion diffusion across cell membranes | 141 | ||
Membrane potentials can be measured with microelectrodes as well as dyes or fluorescent proteins that are voltage sensitive | 143 | ||
Membrane potential is generated by ion gradients | 144 | ||
For mammalian cells, Nernst potentials for ions typically range from −100 mV for K+ to +100 mV for Ca2 | 146 | ||
Currents carried by ions across membranes depend on the concentration of ions on both sides of the membrane, the membrane potential, and the permeability of the membrane to each ion | 146 | ||
Membrane potential depends on ionic concentration gradients and permeabilities | 148 | ||
Electrical Model of a Cell Membrane | 149 | ||
The cell membrane model includes various ionic conductances and electromotive forces in parallel with a capacitor | 149 | ||
The separation of relatively few charges across the bilayer capacitance maintains the membrane potential | 150 | ||
Ionic current is directly proportional to the electrochemical driving force (Ohm’s law) | 150 | ||
Capacitative current is proportional to the rate of voltage change | 151 | ||
A voltage clamp measures currents across cell membranes | 152 | ||
The patch-clamp technique resolves unitary currents through single channel molecules | 154 | ||
Single channel currents sum to produce macroscopic membrane currents | 154 | ||
Single channels can fluctuate between open and closed states | 156 | ||
Molecular Physiology of Ion Channels | 157 | ||
Classes of ion channels can be distinguished on the basis of electrophysiology, pharmacological and physiological ligands, intracellular messengers, and sequence homology | 157 | ||
Electrophysiology | 157 | ||
Pharmacological Ligands | 158 | ||
Physiological Ligands | 158 | ||
Intracellular Messengers | 158 | ||
Sequence Homology | 158 | ||
Many channels are formed by a radially symmetric arrangement of subunits or domains around a central pore | 158 | ||
Gap junction channels are made up of two connexons, each of which has six identical subunits called connexins | 158 | ||
An evolutionary tree called a dendrogram illustrates the relatedness of ion channels | 159 | ||
Hydrophobic domains of channel proteins can predict how these proteins weave through the membrane | 161 | ||
Protein superfamilies, subfamilies, and subtypes are the structural bases of channel diversity | 162 | ||
Connexins | 162 | ||
K+ Channels | 162 | ||
HCN, CNG, and TRP Channels | 162 | ||
NAADP Receptor | 165 | ||
Voltage-Gated Na+ Channels | 165 | ||
Voltage-Gated Ca2+ Channels | 165 | ||
CatSper Channels | 165 | ||
Hv Channels | 165 | ||
Ligand-Gated Channels | 165 | ||
Other Ion Channels | 165 | ||
References | 165 | ||
References | 165.e2 | ||
Books and Reviews | 165.e2 | ||
Journal Articles | 165.e2 | ||
7 Electrical Excitability and Action Potentials | 173 | ||
Mechanisms of Nerve and Muscle Action Potentials | 173 | ||
An action potential is a transient depolarization triggered by a depolarization beyond a threshold | 173 | ||
In contrast to an action potential, a graded response is proportional to stimulus intensity and decays with distance along the axon | 174 | ||
Excitation of a nerve or muscle depends on the product (strength × duration) of the stimulus and on the refractory period | 176 | ||
The action potential arises from changes in membrane conductance to Na+ and K | 176 | ||
The Na+ and K+ currents that flow during the action potential are time and voltage dependent | 177 | ||
Time Dependence of Na+ and K+ Currents | 177 | ||
Voltage Dependence of Na+ and K+ Currents | 178 | ||
Macroscopic Na+ and K+ currents result from the opening and closing of many channels | 180 | ||
The Hodgkin-Huxley model predicts macroscopic currents and the shape of the action potential | 181 | ||
Physiology of Voltage-Gated Channels and Their Relatives | 182 | ||
A large superfamily of structurally related membrane proteins includes voltage-gated and related channels | 182 | ||
Na+ channels generate the rapid initial depolarization of the action potential | 185 | ||
Na+ channels are blocked by neurotoxins and local anesthetics | 187 | ||
Ca2+ channels contribute to action potentials in some cells and also function in electrical and chemical coupling mechanisms | 189 | ||
Ca2+ channels are characterized as L-, T-, P/Q-, N-, and R-type channels on the basis of kinetic properties and inhibitor sensitivity | 190 | ||
K+ channels determine resting potential and regulate the frequency and termination of action potentials | 193 | ||
The Kv (or Shaker-related) family of K+ channels mediates both the delayed outward-rectifier current and the transient A-type current | 193 | ||
Two families of KCa K+ channels mediate Ca2+-activated K+ currents | 196 | ||
The Kir K+ channels mediate inward-rectifier K+ currents, and K2P channels may sense stress | 196 | ||
Propagation of Action Potentials | 199 | ||
The propagation of electrical signals in the nervous system involves local current loops | 199 | ||
Myelin improves the efficiency with which axons conduct action potentials | 199 | ||
The cable properties of the membrane and cytoplasm determine the velocity of signal propagation | 201 | ||
References | 203 | ||
References | 203.e1 | ||
Books and Reviews | 203.e1 | ||
Journal Articles | 203.e1 | ||
8 Synaptic Transmission and the Neuromuscular Junction | 204 | ||
Mechanisms of Synaptic Transmission | 204 | ||
Electrical continuity between cells is established by electrical or chemical synapses | 204 | ||
Electrical synapses directly link the cytoplasm of adjacent cells | 205 | ||
Chemical synapses use neurotransmitters to provide electrical continuity between adjacent cells | 206 | ||
Neurotransmitters can activate ionotropic or metabotropic receptors | 206 | ||
Synaptic Transmission at the Neuromuscular Junction | 208 | ||
Neuromuscular junctions are specialized synapses between motor neurons and skeletal muscle | 208 | ||
ACh activates nicotinic AChRs to produce an excitatory end-plate current | 210 | ||
The nicotinic AChR is a member of the pentameric Cys-loop receptor family of ligand-gated ion channels | 212 | ||
Activation of AChR channels requires binding of two ACh molecules | 213 | ||
Miniature EPPs reveal the quantal nature of transmitter release from the presynaptic terminals | 214 | ||
Direct sensing of extracellular transmitter also shows quantal release of transmitter | 215 | ||
Synaptic vesicles package, store, and deliver neurotransmitters | 217 | ||
Neurotransmitter release occurs by exocytosis of synaptic vesicles | 219 | ||
Re-uptake or cleavage of the neurotransmitter terminates its action | 221 | ||
Toxins and Drugs Affecting Synaptic Transmission | 222 | ||
Guanidinium neurotoxins such as tetrodotoxin prevent depolarization of the nerve terminal, whereas dendrotoxins inhibit repolarization | 222 | ||
ω-Conotoxin blocks Ca2+ channels that mediate Ca2+ influx into nerve terminals, inhibiting synaptic transmission | 224 | ||
Bacterial toxins such as tetanus and botulinum toxins cleave proteins involved in exocytosis, preventing fusion of synaptic vesicles | 224 | ||
Both agonists and antagonists of the nicotinic AChR can prevent synaptic transmission | 225 | ||
Inhibitors of AChE prolong and magnify the EPP | 226 | ||
References | 227 | ||
References | 227.e2 | ||
Books and Reviews | 227.e2 | ||
Journal Articles | 227.e2 | ||
9 Cellular Physiology of Skeletal, Cardiac, and Smooth Muscle | 228 | ||
Skeletal Muscle | 228 | ||
Contraction of skeletal muscle is initiated by motor neurons that innervate motor units | 228 | ||
Action potentials propagate from the sarcolemma to the interior of muscle fibers along the transverse tubule network | 229 | ||
Depolarization of the T-tubule membrane results in Ca2+ release from the SR at the triad | 229 | ||
Striations of skeletal muscle fibers correspond to ordered arrays of thick and thin filaments within myofibrils | 232 | ||
Thin and thick filaments are supramolecular assemblies of protein subunits | 233 | ||
Thin Filaments | 233 | ||
Thick Filaments | 233 | ||
During the cross-bridge cycle, contractile proteins convert the energy of ATP hydrolysis into mechanical energy | 234 | ||
An increase in [Ca2+]i triggers contraction by removing the inhibition of cross-bridge cycling | 236 | ||
Termination of contraction requires re-uptake of Ca2+ into the SR | 237 | ||
Muscle contractions produce force under isometric conditions and force with shortening under isotonic conditions | 237 | ||
Muscle length influences tension development by determining the degree of overlap between actin and myosin filaments | 238 | ||
At higher loads, the velocity of shortening is lower because more cross-bridges are simultaneously active | 240 | ||
In a single skeletal muscle fiber, the force developed may be increased by summing multiple twitches in time | 241 | ||
In a whole skeletal muscle, the force developed may be increased by summing the contractions of multiple fibers | 241 | ||
Cardiac Muscle | 242 | ||
Action potentials propagate between adjacent cardiac myocytes through gap junctions | 242 | ||
Cardiac contraction requires Ca2+ entry through L-type Ca2+ channels | 242 | ||
Cross-bridge cycling and termination of cardiac muscle contraction are similar to the events in skeletal muscle | 243 | ||
In cardiac muscle, increasing the entry of Ca2+ enhances the contractile force | 243 | ||
Smooth Muscle | 243 | ||
Smooth muscles may contract in response to synaptic transmission or electrical coupling | 243 | ||
Action potentials of smooth muscles may be brief or prolonged | 244 | ||
Some smooth-muscle cells spontaneously generate either pacemaker currents or slow waves | 244 | ||
Some smooth muscles contract without action potentials | 246 | ||
In smooth muscle, both entry of extracellular Ca2+ and intracellular Ca2+ spark activate contraction | 246 | ||
Ca2+ Entry via Voltage-Gated Channels | 247 | ||
Ca2+ Release from the SR | 247 | ||
Ca2+ Entry through Store-Operated Ca2+ Channels (SOCs) | 247 | ||
Ca2+-dependent phosphorylation of the myosin regulatory light chain activates cross-bridge cycling in smooth muscle | 247 | ||
Termination of smooth-muscle contraction requires dephosphorylation of myosin light chain | 248 | ||
Smooth-muscle contraction may also occur independently of increases in [Ca2+]i | 248 | ||
In smooth muscle, increases in both [Ca2+]i and the Ca2+ sensitivity of the contractile apparatus enhance contractile force | 249 | ||
Smooth muscle maintains high force at low energy consumption | 249 | ||
Diversity among Muscles | 249 | ||
Skeletal muscle is composed of slow-twitch and fast-twitch fibers | 249 | ||
The properties of cardiac cells vary with location in the heart | 250 | ||
The properties of smooth-muscle cells differ markedly among tissues and may adapt with time | 250 | ||
Smooth-muscle cells express a wide variety of neurotransmitter and hormone receptors | 251 | ||
References | 251 | ||
References | 251.e1 | ||
Books and Reviews | 251.e1 | ||
Journal Articles | 251.e1 | ||
III The Nervous System | 253 | ||
10 Organization of the Nervous System | 254 | ||
The nervous system can be divided into central, peripheral, and autonomic nervous systems | 254 | ||
Each area of the nervous system has unique nerve cells and a different function | 254 | ||
Cells of the Nervous System | 255 | ||
The neuron doctrine first asserted that the nervous system is composed of many individual signaling units—the neurons | 255 | ||
Nerve cells have four specialized regions: cell body, dendrites, axon, and presynaptic terminals | 255 | ||
Cell Body | 255 | ||
Dendrites | 255 | ||
Axon | 255 | ||
Presynaptic Terminals | 256 | ||
The cytoskeleton helps compartmentalize the neuron and also provides the tracks along which material travels between different parts of the neuron | 257 | ||
Fast Axoplasmic Transport | 257 | ||
Fast Retrograde Transport | 259 | ||
Slow Axoplasmic Transport | 259 | ||
Neurons can be classified on the basis of their axonal projection, their dendritic geometry, and the number of processes emanating from the cell body | 259 | ||
Axonal Projection | 259 | ||
Dendritic Geometry | 259 | ||
Number of Processes | 259 | ||
Glial cells provide a physiological environment for neurons | 259 | ||
Development of Neurons and Glial Cells | 261 | ||
Neurons differentiate from the neuroectoderm | 261 | ||
Neurons and glial cells originate from cells in the proliferating germinal matrix near the ventricles | 263 | ||
Neurons migrate to their correct anatomical position in the brain with the help of adhesion molecules | 267 | ||
Neurons do not regenerate | 267 | ||
Neurons | 267 | ||
Axons | 267 | ||
Glia | 269 | ||
Subdivisions of the Nervous System | 269 | ||
The CNS consists of the telencephalon, cerebellum, diencephalon, midbrain, pons, medulla, and spinal cord | 269 | ||
Telencephalon | 269 | ||
Cerebellum | 270 | ||
Diencephalon | 270 | ||
Brainstem (Midbrain, Pons, and Medulla) | 270 | ||
Spinal Cord | 271 | ||
The PNS comprises the cranial and spinal nerves, their associated sensory ganglia, and various sensory receptors | 271 | ||
The ANS innervates effectors that are not under voluntary control | 273 | ||
References | 274 | ||
References | 274.e1 | ||
Books and Reviews | 274.e1 | ||
Journal Articles | 274.e1 | ||
11 The Neuronal Microenvironment | 275 | ||
Extracellular fluid in the brain provides a highly regulated environment for central nervous system neurons | 275 | ||
The brain is physically and metabolically fragile | 275 | ||
Cerebrospinal Fluid | 275 | ||
CSF fills the ventricles and subarachnoid space | 275 | ||
The brain floats in CSF, which acts as a shock absorber | 278 | ||
The choroid plexuses secrete CSF into the ventricles, and the arachnoid granulations absorb it | 278 | ||
The epithelial cells of the choroid plexus secrete the CSF | 279 | ||
Brain Extracellular Space | 282 | ||
Neurons, glia, and capillaries are packed tightly together in the CNS | 282 | ||
The CSF communicates freely with the BECF, which stabilizes the composition of the neuronal microenvironment | 282 | ||
The ion fluxes that accompany neural activity cause large changes in extracellular ion concentration | 284 | ||
The Blood-Brain Barrier | 284 | ||
The blood-brain barrier prevents some blood constituents from entering the brain extracellular space | 284 | ||
Continuous tight junctions link brain capillary endothelial cells | 285 | ||
Uncharged and lipid-soluble molecules more readily pass through the blood-brain barrier | 286 | ||
Transport by capillary endothelial cells contributes to the blood-brain barrier | 286 | ||
Glial Cells | 287 | ||
Glial cells constitute half the volume of the brain and outnumber neurons | 287 | ||
Astrocytes supply fuel to neurons in the form of lactic acid | 287 | ||
Astrocytes are predominantly permeable to K+ and also help regulate [K+]o | 289 | ||
Gap junctions couple astrocytes to one another, allowing diffusion of small solutes | 289 | ||
Astrocytes synthesize neurotransmitters, take them up from the extracellular space, and have neurotransmitter receptors | 290 | ||
Astrocytes secrete trophic factors that promote neuronal survival and synaptogenesis | 292 | ||
Astrocytic endfeet modulate cerebral blood flow | 292 | ||
Oligodendrocytes and Schwann cells make and sustain myelin | 292 | ||
Oligodendrocytes are involved in pH regulation and iron metabolism in the brain | 293 | ||
Microglial cells are the macrophages of the CNS | 294 | ||
References | 294 | ||
References | 294.e1 | ||
Books and Reviews | 294.e1 | ||
Journal Articles | 294.e1 | ||
12 Physiology of Neurons | 295 | ||
Neurons receive, combine, transform, store, and send information | 295 | ||
Neural information flows from dendrite to soma to axon to synapse | 295 | ||
Signal Conduction in Dendrites | 297 | ||
Dendrites attenuate synaptic potentials | 297 | ||
Dendritic membranes have voltage-gated ion channels | 299 | ||
Control of Spiking Patterns in the Soma | 300 | ||
Neurons can transform a simple input into a variety of output patterns | 300 | ||
Intrinsic firing patterns are determined by a variety of ion currents with relatively slow kinetics | 301 | ||
Axonal Conduction | 301 | ||
Axons are specialized for rapid, reliable, and efficient transmission of electrical signals | 301 | ||
Action potentials are usually initiated at the initial segment | 302 | ||
Conduction velocity of a myelinated axon increases linearly with diameter | 302 | ||
Demyelinated axons conduct action potentials slowly, unreliably, or not at all | 304 | ||
References | 306 | ||
References | 306.e1 | ||
Books and Reviews | 306.e1 | ||
Journal Articles | 306.e1 | ||
13 Synaptic Transmission in the Nervous System | 307 | ||
Neuronal Synapses | 307 | ||
The molecular mechanisms of neuronal synapses are similar but not identical to those of the neuromuscular junction | 307 | ||
Presynaptic terminals may contact neurons at the dendrite, soma, or axon and may contain both clear vesicles and dense-core granules | 309 | ||
The postsynaptic membrane contains transmitter receptors and numerous proteins clustered in the postsynaptic density | 310 | ||
Some transmitters are used by diffusely distributed systems of neurons to modulate the general excitability of the brain | 311 | ||
Electrical synapses serve specialized functions in the mammalian nervous system | 314 | ||
Neurotransmitter Systems of the Brain | 314 | ||
Most of the brain’s transmitters are common biochemicals | 315 | ||
Synaptic transmitters can stimulate, inhibit, or modulate the postsynaptic neuron | 318 | ||
Excitatory Synapses | 318 | ||
Inhibitory Synapses | 319 | ||
Modulatory Synapses | 319 | ||
G proteins may affect ion channels directly, or indirectly through second messengers | 320 | ||
Signaling cascades allow amplification, regulation, and a long duration of transmitter responses | 321 | ||
Neurotransmitters may have both convergent and divergent effects | 322 | ||
Fast Amino Acid–Mediated Synapses in the CNS | 322 | ||
Most EPSPs in the brain are mediated by two types of glutamate-gated channels | 323 | ||
Most IPSPs in the brain are mediated by the GABAA receptor, which is activated by several classes of drugs | 325 | ||
The ionotropic receptors for ACh, serotonin, GABA, and glycine belong to the superfamily of ligand-gated/pentameric channels | 326 | ||
Most neuronal synapses release a very small number of transmitter quanta with each action potential | 327 | ||
When multiple transmitters colocalize to the same synapse, the exocytosis of large vesicles requires high-frequency stimulation | 327 | ||
Plasticity of Central Synapses | 328 | ||
Use-dependent changes in synaptic strength underlie many forms of learning | 328 | ||
Short-term synaptic plasticity usually reflects presynaptic changes | 328 | ||
Long-term potentiation in the hippocampus may last for days or weeks | 329 | ||
Long-term depression exists in multiple forms | 331 | ||
Long-term depression in the cerebellum may be important for motor learning | 331 | ||
References | 333 | ||
References | 333.e1 | ||
Books and Reviews | 333.e1 | ||
Journal Articles | 333.e1 | ||
14 The Autonomic Nervous System | 334 | ||
Organization of the Visceral Control System | 334 | ||
The ANS has sympathetic, parasympathetic, and enteric divisions | 334 | ||
Sympathetic preganglionic neurons originate from spinal segments T1 to L3 and synapse with postganglionic neurons in paravertebral or prevertebral ganglia | 335 | ||
Preganglionic Neurons | 335 | ||
Paravertebral Ganglia | 335 | ||
Prevertebral Ganglia | 336 | ||
Postganglionic Neurons | 336 | ||
Cranial Nerves III, VII, and IX | 338 | ||
Cranial Nerve X | 339 | ||
Sacral Nerves | 339 | ||
The visceral control system also has an important afferent limb | 339 | ||
The enteric division is a self-contained nervous system of the GI tract and receives sympathetic and parasympathetic input | 339 | ||
Synaptic Physiology of the Autonomic Nervous System | 340 | ||
The sympathetic and parasympathetic divisions have opposite effects on most visceral targets | 340 | ||
All preganglionic neurons—both sympathetic and parasympathetic—release acetylcholine and stimulate N2 nicotinic receptors on postganglionic neurons | 341 | ||
All postganglionic parasympathetic neurons release ACh and stimulate muscarinic receptors on visceral targets | 341 | ||
Most postganglionic sympathetic neurons release norepinephrine onto visceral targets | 342 | ||
Postganglionic sympathetic and parasympathetic neurons often have muscarinic as well as nicotinic receptors | 343 | ||
Nonclassic transmitters can be released at each level of the ANS | 344 | ||
Two of the most unusual nonclassic neurotransmitters, ATP and nitric oxide, were first identified in the ANS | 345 | ||
ATP | 346 | ||
Nitric Oxide | 346 | ||
Central Nervous System Control of the Viscera | 347 | ||
Sympathetic output can be massive and nonspecific, as in the fight-or-flight response, or selective for specific target organs | 347 | ||
Parasympathetic neurons participate in many simple involuntary reflexes | 348 | ||
A variety of brainstem nuclei provide basic control of the ANS | 348 | ||
The forebrain can modulate autonomic output, and reciprocally, visceral sensory input integrated in the brainstem can influence or even overwhelm the forebrain | 348 | ||
CNS control centers oversee visceral feedback loops and orchestrate a feed-forward response to meet anticipated needs | 349 | ||
The ANS has multiple levels of reflex loops | 350 | ||
References | 352 | ||
References | 352.e1 | ||
Books and Reviews | 352.e1 | ||
Journal Articles | 352.e1 | ||
15 Sensory Transduction | 353 | ||
Sensory receptors convert environmental energy into neural signals | 353 | ||
Sensory transduction uses adaptations of common molecular signaling mechanisms | 353 | ||
Sensory transduction requires detection and amplification, usually followed by a local receptor potential | 353 | ||
Chemoreception | 354 | ||
Chemoreceptors are ubiquitous, diverse, and evolutionarily ancient | 354 | ||
Taste receptors are modified epithelial cells, whereas olfactory receptors are neurons | 354 | ||
Taste Receptor Cells | 354 | ||
Olfactory Receptor Cells | 354 | ||
Complex flavors are derived from a few basic types of taste receptors, with contributions from sensory receptors of smell, temperature, texture, and pain | 356 | ||
Taste transduction involves many types of molecular signaling systems | 356 | ||
Salty | 357 | ||
Sour | 357 | ||
Sweet | 358 | ||
Bitter | 358 | ||
Amino Acids | 358 | ||
Olfactory transduction involves specific receptors, G protein–coupled signaling, and a cyclic nucleotide–gated ion channel | 358 | ||
Visual Transduction | 359 | ||
The optical components of the eye collect light and focus it onto the retina | 360 | ||
The retina is a small, displaced part of the CNS | 363 | ||
There are three primary types of photoreceptors: rods, cones, and intrinsically photosensitive ganglion cells | 363 | ||
Rods and cones hyperpolarize in response to light | 365 | ||
Rhodopsin is a G protein–coupled “receptor” for light | 367 | ||
The eye uses a variety of mechanisms to adapt to a wide range of light levels | 368 | ||
Color vision depends on the different spectral sensitivities of the three types of cones | 369 | ||
The ipRGCs have unique properties and functions | 370 | ||
Vestibular and Auditory Transduction: Hair Cells | 371 | ||
Bending the stereovilli of hair cells along one axis causes cation channels to open or to close | 372 | ||
The otolithic organs (saccule and utricle) detect the orientation and linear acceleration of the head | 374 | ||
The semicircular canals detect the angular acceleration of the head | 375 | ||
The outer and middle ears collect and condition air pressure waves for transduction within the inner ear | 376 | ||
Outer Ear | 376 | ||
Middle Ear | 376 | ||
The cochlea is a spiral of three parallel, fluid-filled tubes | 377 | ||
Inner hair cells transduce sound, whereas the active movements of outer hair cells amplify the signal | 378 | ||
The frequency sensitivity of auditory hair cells depends on their position along the basilar membrane of the cochlea | 380 | ||
Somatic Sensory Receptors, Proprioception, and Pain | 383 | ||
A variety of sensory endings in the skin transduce mechanical, thermal, and chemical stimuli | 383 | ||
Mechanoreceptors in the skin provide sensitivity to specific stimuli such as vibration and steady pressure | 383 | ||
Separate thermoreceptors detect warmth and cold | 385 | ||
Nociceptors are specialized sensory endings that transduce painful stimuli | 387 | ||
Muscle spindles sense changes in the length of skeletal muscle fibers, whereas Golgi tendon organs gauge the muscle’s force | 388 | ||
References | 389 | ||
References | 389.e1 | ||
Books and Reviews | 389.e1 | ||
Journal Articles | 389.e1 | ||
16 Circuits of the Central Nervous System | 390 | ||
Elements of Neural Circuits | 390 | ||
Neural circuits process sensory information, generate motor output, and create spontaneous activity | 390 | ||
Nervous systems have several levels of organization | 390 | ||
Most local circuits have three elements: input axons, interneurons, and projection (output) neurons | 391 | ||
Simple, Stereotyped Responses: Spinal Reflex Circuits | 392 | ||
Passive stretching of a skeletal muscle causes a reflexive contraction of that same muscle and relaxation of the antagonist muscles | 392 | ||
Force applied to the Golgi tendon organ regulates muscle contractile strength | 394 | ||
Noxious stimuli can evoke complex reflexive movements | 394 | ||
Spinal reflexes are strongly influenced by control centers within the brain | 394 | ||
Rhythmic Activity: Central Pattern Generators | 396 | ||
Central pattern generators in the spinal cord can create a complex motor program even without sensory feedback | 396 | ||
Pacemaker cells and synaptic interconnections both contribute to central pattern generation | 397 | ||
Central pattern generators in the spinal cord take advantage of sensory feedback, interconnections among spinal segments, and interactions with brainstem control centers | 398 | ||
Spatial Representations: Sensory and Motor Maps in the Brain | 399 | ||
The nervous system contains maps of sensory and motor information | 399 | ||
The cerebral cortex has multiple visuotopic maps | 399 | ||
Maps of somatic sensory information magnify some parts of the body more than others | 400 | ||
The cerebral cortex has a motor map that is adjacent to and well aligned with the somatosensory map | 402 | ||
Sensory and motor maps are fuzzy and plastic | 403 | ||
Temporal Representations: Time-Measuring Circuits | 405 | ||
To localize sound, the brain compares the timing and intensity of input to the ears | 405 | ||
The brain measures interaural timing by a combination of neural delay lines and coincidence detectors | 405 | ||
References | 407 | ||
References | 407.e1 | ||
Books and Reviews | 407.e1 | ||
Journal Articles | 407.e1 | ||
IV The Cardiovascular System | 409 | ||
17 Organization of the Cardiovascular System | 410 | ||
Elements of the Cardiovascular System | 410 | ||
The circulation is an evolutionary consequence of body size | 410 | ||
The heart is a dual pump that drives the blood in two serial circuits: the systemic and the pulmonary circulations | 410 | ||
Hemodynamics | 412 | ||
Blood flow is driven by a constant pressure head across variable resistances | 412 | ||
Blood pressure is always measured as a pressure difference between two points | 412 | ||
Total blood flow, or cardiac output, is the product (heart rate) × (stroke volume) | 414 | ||
Flow in an idealized vessel increases with the fourth power of radius (Poiseuille equation) | 415 | ||
Viscous resistance to flow is proportional to the viscosity of blood but does not depend on properties of the blood vessel walls | 415 | ||
The viscosity of blood is a measure of the internal slipperiness between layers of fluid | 415 | ||
How Blood Flows | 416 | ||
Blood flow is laminar | 416 | ||
Pressure and flow oscillate with each heartbeat between maximum systolic and minimum diastolic values | 417 | ||
Origins of Pressure in the Circulation | 418 | ||
Gravity causes a hydrostatic pressure difference when there is a difference in height | 418 | ||
Low compliance of a vessel causes the transmural pressure to increase when the vessel blood volume is increased | 419 | ||
The viscous resistance of blood causes an axial pressure difference when there is flow | 419 | ||
The inertia of the blood and vessels causes pressure to decrease when the velocity of blood flow increases | 420 | ||
How to Measure Blood Pressure, Blood Flow, and Cardiac Volumes | 420 | ||
Blood pressure can be measured directly by puncturing the vessel | 420 | ||
Blood pressure can be measured indirectly by use of a sphygmomanometer | 421 | ||
Blood flow can be measured directly by electromagnetic and ultrasound flowmeters | 422 | ||
Invasive Methods | 422 | ||
Noninvasive Methods | 423 | ||
Cardiac output can be measured indirectly by the Fick method, which is based on the conservation of mass | 423 | ||
Cardiac output can be measured indirectly by dilution methods | 424 | ||
Regional blood flow can be measured indirectly by “clearance” methods | 426 | ||
Ventricular dimensions, ventricular volumes, and volume changes can be measured by angiography and echocardiography | 426 | ||
References | 428 | ||
References | 428.e2 | ||
Books and Reviews | 428.e2 | ||
Journal Articles | 428.e2 | ||
18 Blood | 429 | ||
Blood Composition | 429 | ||
Whole blood is a suspension of cellular elements in plasma | 429 | ||
Bone marrow is the source of most blood cells | 431 | ||
RBCs are mainly composed of hemoglobin | 434 | ||
Leukocytes defend against infections | 435 | ||
Neutrophils | 435 | ||
Eosinophils | 435 | ||
Basophils | 435 | ||
Lymphocytes | 435 | ||
Monocytes | 435 | ||
Platelets are nucleus-free fragments | 435 | ||
Blood Viscosity | 436 | ||
Whole blood has an anomalous viscosity | 436 | ||
Blood viscosity increases with the hematocrit and the fibrinogen plasma concentration | 437 | ||
Fibrinogen | 437 | ||
Hematocrit | 437 | ||
Vessel Radius | 437 | ||
Velocity of Flow | 438 | ||
Temperature | 438 | ||
Hemostasis and Fibrinolysis | 439 | ||
Platelets can plug holes in small vessels | 439 | ||
Adhesion | 439 | ||
Activation | 440 | ||
Aggregation | 440 | ||
A controlled cascade of proteolysis creates a blood clot | 440 | ||
Intrinsic Pathway (Surface Contact Activation) | 442 | ||
Extrinsic Pathway (Tissue Factor Activation) | 442 | ||
Common Pathway | 442 | ||
Coagulation as a Connected Diagram | 444 | ||
Anticoagulants keep the clotting network in check | 444 | ||
Paracrine Factors | 444 | ||
Anticoagulant Factors | 444 | ||
Fibrinolysis breaks up clots | 445 | ||
References | 446 | ||
References | 446.e1 | ||
Books and Reviews | 446.e1 | ||
Journal Articles | 446.e1 | ||
19 Arteries and Veins | 447 | ||
Arterial Distribution and Venous Collection Systems | 447 | ||
Physical properties of vessels closely follow the level of branching in the circuit | 447 | ||
Most of the blood volume resides in the systemic veins | 448 | ||
The intravascular pressures along the systemic circuit are higher than those along the pulmonary circuit | 450 | ||
Under normal conditions, the steepest pressure drop in the systemic circulation occurs in arterioles, the site of greatest vascular resistance | 451 | ||
Local intravascular pressure depends on the distribution of vascular resistance | 451 | ||
Elastic Properties of Blood Vessels | 452 | ||
Blood vessels are elastic tubes | 452 | ||
Because of the elastic properties of vessels, the pressure-flow relationship of passive vascular beds is nonlinear | 453 | ||
Contraction of smooth muscle halts blood flow when driving pressure falls below the critical closing pressure | 454 | ||
Elastic and collagen fibers determine the distensibility and compliance of vessels | 454 | ||
Differences in compliance cause arteries to act as resistors and veins to act as capacitors | 455 | ||
Laplace’s law describes how tension in the vessel wall increases with transmural pressure | 455 | ||
The vascular wall is adapted to withstand wall tension, not transmural pressure | 457 | ||
Elastin and collagen separately contribute to the wall tension of vessels | 458 | ||
Aging reduces the distensibility of arteries | 458 | ||
Active tension from smooth-muscle activity adds to the elastic tension of vessels | 459 | ||
Elastic tension helps stabilize vessels under vasomotor control | 460 | ||
References | 460 | ||
References | 460.e1 | ||
Books and Reviews | 460.e1 | ||
Journal Articles | 460.e1 | ||
20 The Microcirculation | 461 | ||
The microcirculation serves both nutritional and non-nutritional roles | 461 | ||
The microcirculation extends from the arterioles to the venules | 461 | ||
Capillary Exchange of Solutes | 463 | ||
The exchange of O2 and CO2 across capillaries depends on the diffusional properties of the surrounding tissue | 463 | ||
The O2 extraction ratio of a whole organ depends primarily on blood flow and metabolic demand | 464 | ||
According to Fick’s law, the diffusion of small water-soluble solutes across a capillary wall depends on both the permeability and the concentration gradient | 464 | ||
The whole-organ extraction ratio for small hydrophilic solutes provides an estimate of the solute permeability of capillaries | 465 | ||
Small polar molecules have a relatively low permeability because they can traverse the capillary wall only by diffusing through water-filled pores (small-pore effect) | 466 | ||
The exchange of macromolecules across capillaries can occur by transcytosis (large-pore effect) | 467 | ||
Capillary Exchange of Water | 467 | ||
Fluid transfer across capillaries is convective and depends on net hydrostatic and osmotic forces (i.e., Starling forces) | 467 | ||
Capillary blood pressure (Pc) falls from ~35 mm Hg at the arteriolar end to ~15 mm Hg at the venular end | 469 | ||
Arteriolar (Pa) and Venular (Pv) Pressure | 469 | ||
Location | 469 | ||
Time | 469 | ||
Gravity | 469 | ||
Interstitial fluid pressure (Pif) is slightly negative, except in encapsulated organs | 469 | ||
Capillary colloid osmotic pressure (πc), which reflects the presence of plasma proteins, is ~25 mm Hg | 470 | ||
Interstitial fluid colloid osmotic pressure (πif) varies between 0 and 10 mm Hg among different organs | 470 | ||
The Starling principle predicts ultrafiltration at the arteriolar end and absorption at the venular end of most capillary beds | 471 | ||
For continuous capillaries, the endothelial barrier for fluid exchange is more complex than considered by Starling | 472 | ||
Lymphatics | 474 | ||
Lymphatics return excess interstitial fluid to the blood | 474 | ||
Flow in Initial Lymphatics | 475 | ||
Flow in Collecting Lymphatics | 475 | ||
Transport of Proteins and Cells | 475 | ||
The circulation of extracellular fluids involves three convective loops: blood, interstitial fluid, and lymph | 476 | ||
Regulation of the Microcirculation | 477 | ||
The active contraction of vascular smooth muscle regulates precapillary resistance, which controls capillary blood flow | 477 | ||
Contraction of Vascular Smooth Muscle | 477 | ||
Relaxation of Vascular Smooth Muscle | 477 | ||
Tissue metabolites regulate local blood flow in specific vascular beds, independently of the systemic regulation | 477 | ||
The endothelium of capillary beds is the source of several vasoactive compounds, including nitric oxide, endothelium-derived hyperpolarizing factor, and endothelin | 480 | ||
Nitric Oxide | 480 | ||
Endothelium-Derived Hyperpolarizing Factor | 480 | ||
Prostacyclin (Prostaglandin I2) | 480 | ||
Endothelins | 480 | ||
Thromboxane A2 | 480 | ||
Other Endothelial Factors | 481 | ||
Autoregulation stabilizes blood flow despite large fluctuations in systemic arterial pressure | 481 | ||
Blood vessels proliferate in response to growth factors by a process known as angiogenesis | 481 | ||
Promoters of Vessel Growth | 481 | ||
Inhibitors of Vessel Growth | 482 | ||
References | 482 | ||
References | 482.e2 | ||
Books and Reviews | 482.e2 | ||
Journal Articles | 482.e2 | ||
21 Cardiac Electrophysiology and the Electrocardiogram | 483 | ||
Electrophysiology of Cardiac Cells | 483 | ||
The cardiac action potential starts in specialized muscle cells of the sinoatrial node and then propagates in an orderly fashion throughout the heart | 483 | ||
The cardiac action potential conducts from cell to cell via gap junctions | 483 | ||
Cardiac action potentials have as many as five distinctive phases | 484 | ||
The Na+ current is the largest current in the heart | 485 | ||
The Ca2+ current in the heart passes primarily through L-type Ca2+ channels | 488 | ||
The repolarizing K+ current turns on slowly | 488 | ||
Early Outward K+ Current (A-type Current) | 488 | ||
G Protein–Activated K+ Current | 488 | ||
KATP Current | 488 | ||
The If current is mediated by a nonselective cation channel | 488 | ||
Different cardiac tissues uniquely combine ionic currents to produce distinctive action potentials | 488 | ||
The SA node is the primary pacemaker of the heart | 489 | ||
The Concept of Pacemaker Activity | 489 | ||
SA Node | 489 | ||
AV Node | 489 | ||
Purkinje Fibers | 490 | ||
Atrial and ventricular myocytes fire action potentials but do not have pacemaker activity | 490 | ||
Atrial Muscle | 490 | ||
Ventricular Muscle | 490 | ||
Acetylcholine and catecholamines modulate pacemaker activity, conduction velocity, and contractility | 491 | ||
Acetylcholine | 492 | ||
Catecholamines | 492 | ||
The Electrocardiogram | 493 | ||
An ECG generally includes five waves | 493 | ||
A pair of ECG electrodes defines a lead | 493 | ||
The Limb Leads | 494 | ||
The Precordial Leads | 494 | ||
A simple two-cell model can explain how a simple ECG can arise | 496 | ||
Cardiac Arrhythmias | 496 | ||
Conduction abnormalities are a major cause of arrhythmias | 497 | ||
Partial (or Incomplete) Conduction Block | 502 | ||
Complete Conduction Block | 502 | ||
Re-Entry | 502 | ||
Accessory Conduction Pathways | 504 | ||
Fibrillation | 505 | ||
Altered automaticity can originate from the sinus node or from an ectopic locus | 505 | ||
Depolarization-Dependent Triggered Activity | 505 | ||
Long QT Syndrome | 506 | ||
Ca2+ overload and metabolic changes can also cause arrhythmias | 506 | ||
Ca2+ Overload | 506 | ||
Metabolism-Dependent Conduction Changes | 506 | ||
Electromechanical Dissociation | 506 | ||
References | 506 | ||
References | 506.e1 | ||
Books and Reviews | 506.e1 | ||
Journal Articles | 506.e1 | ||
22 The Heart as a Pump | 507 | ||
The Cardiac Cycle | 507 | ||
The closing and opening of the cardiac valves define four phases of the cardiac cycle | 507 | ||
Changes in ventricular volume, pressure, and flow accompany the four phases of the cardiac cycle N22-1 | 508 | ||
Diastasis Period (Middle of Phase 1) | 508 | ||
Atrial Contraction (End of Phase 1) | 508 | ||
Isovolumetric Contraction (Phase 2) | 508 | ||
Ejection or Outflow (Phase 3) | 509 | ||
Isovolumetric Relaxation (Phase 4) | 509 | ||
Rapid Ventricular Filling Period (Beginning of Phase 1) | 510 | ||
The ECG, phonocardiogram, and echocardiogram all follow the cyclic pattern of the cardiac cycle | 510 | ||
Aortic Blood Flow | 510 | ||
Jugular Venous Pulse | 510 | ||
Electrocardiogram | 510 | ||
Phonocardiogram and Heart Sounds | 511 | ||
Echocardiogram | 511 | ||
The cardiac cycle causes flow waves in the aorta and peripheral vessels | 511 | ||
Aortic Arch | 513 | ||
Thoracic-Abdominal Aorta and Large Arteries | 513 | ||
The cardiac cycle also causes pressure waves in the aorta and peripheral vessels | 513 | ||
Terminal Arteries and Arterioles | 513 | ||
Capillaries | 513 | ||
Distortion of pressure waves is the result of their propagation along the arterial tree | 513 | ||
Effect of Frequency on Wave Velocity and Damping | 515 | ||
Effect of Wall Stiffness on Wave Velocity | 515 | ||
Pressure waves in veins do not originate from arterial waves | 515 | ||
Effect of the Cardiac Cycle | 516 | ||
Effect of the Respiratory Cycle | 516 | ||
Effect of Skeletal Muscle Contraction (“Muscle Pump”) | 516 | ||
Cardiac Dynamics | 517 | ||
The right ventricle contracts like a bellows, whereas the left ventricle contracts like a hand squeezing a tube of toothpaste | 517 | ||
The right atrium contracts before the left, but the left ventricle contracts before the right | 517 | ||
Atrial Contraction | 517 | ||
Initiation of Ventricular Contraction | 517 | ||
Ventricular Ejection | 517 | ||
Ventricular Relaxation | 519 | ||
Measurements of ventricular volumes, pressures, and flows allow clinicians to judge cardiac performance | 519 | ||
Definitions of Cardiac Volumes | 519 | ||
Measurements of Cardiac Volumes | 519 | ||
Measurement of Ventricular Pressures | 519 | ||
Measurement of Flows | 519 | ||
The pressure-volume loop of a ventricle illustrates the ejection work of the ventricle | 519 | ||
Segment AB | 520 | ||
Segment BC | 520 | ||
Segment CD | 520 | ||
Segment DE | 520 | ||
Segment EF | 520 | ||
Segment FA | 520 | ||
The “pumping work” done by the heart accounts for a small fraction of the total energy the heart consumes | 520 | ||
From Contractile Filaments to a Regulated Pump | 522 | ||
The entry of Ca2+ from the outside triggers Ca2+-induced Ca2+ release from the sarcoplasmic reticulum | 522 | ||
A global rise in [Ca2+]i initiates contraction of cardiac myocytes | 522 | ||
Phosphorylation of phospholamban and of troponin I speeds cardiac muscle relaxation | 522 | ||
Extrusion of Ca2+ into the ECF | 523 | ||
Reuptake of Ca2+ by the SR | 523 | ||
Uptake of Ca2+ by Mitochondria | 524 | ||
Dissociation of Ca2+ from Troponin C | 524 | ||
The overlap of thick and thin filaments cannot explain the unusual shape of the cardiac length-tension diagram | 524 | ||
Starling’s law states that a greater fiber length (i.e., greater ventricular volume) causes the heart to deliver more mechanical energy | 524 | ||
The velocity of cardiac muscle shortening falls when the contraction occurs against a greater opposing force (or pressure) or at a shorter muscle length (or lower volume) | 526 | ||
Increases in heart rate enhance myocardial tension | 528 | ||
Contractility is an intrinsic measure of cardiac performance | 528 | ||
Effect of Changes in Contractility | 530 | ||
Effect of Changes in Preload (i.e., Initial Sarcomere Length) | 530 | ||
Effect of Changes in Afterload | 530 | ||
Positive inotropic agents increase myocardial contractility by raising [Ca2+]i | 530 | ||
Positive Inotropic Agents | 530 | ||
Negative Inotropic Agents | 530 | ||
References | 532 | ||
References | 532.e1 | ||
Books and Reviews | 532.e1 | ||
Journal Articles | 532.e1 | ||
23 Regulation of Arterial Pressure and Cardiac Output | 533 | ||
Short-Term Regulation of Arterial Pressure | 533 | ||
Systemic mean arterial blood pressure is the principal variable that the cardiovascular system controls | 533 | ||
Neural reflexes mediate the short-term regulation of mean arterial blood pressure | 533 | ||
High-pressure baroreceptors at the carotid sinus and aortic arch are stretch receptors that sense changes in arterial pressure | 534 | ||
Increased arterial pressure raises the firing rate of afferent baroreceptor nerves | 536 | ||
The medulla coordinates afferent baroreceptor signals | 537 | ||
The efferent pathways of the baroreceptor response include both sympathetic and parasympathetic divisions of the autonomic nervous system | 537 | ||
Sympathetic Efferents | 537 | ||
Parasympathetic Efferents | 539 | ||
The principal effectors in the neural control of arterial pressure are the heart, the arteries, the veins, and the adrenal medulla | 539 | ||
Sympathetic Input to the Heart (Cardiac Nerves) | 539 | ||
Parasympathetic Input to the Heart (Vagus Nerve) | 539 | ||
Sympathetic Input to Blood Vessels (Vasoconstrictor Response) | 539 | ||
Parasympathetic Input to Blood Vessels (Vasodilator Response) | 539 | ||
Sympathetic Input to Blood Vessels in Skeletal Muscle (Vasodilator Response) | 539 | ||
Adrenal Medulla | 539 | ||
The unique combination of agonists and receptors determines the end response in cardiac and vascular effector cells | 542 | ||
Adrenergic Receptors in the Heart | 542 | ||
Cholinergic Receptors in the Heart | 542 | ||
Adrenergic Receptors in Blood Vessels | 542 | ||
Cholinergic Receptors in or near Blood Vessels | 543 | ||
Nonadrenergic, Noncholinergic Receptors in Blood Vessels | 543 | ||
The medullary cardiovascular center tonically maintains blood pressure and is under the control of higher brain centers | 543 | ||
Secondary neural regulation of arterial blood pressure depends on chemoreceptors | 544 | ||
Carotid Bodies | 545 | ||
Aortic Bodies | 545 | ||
Afferent Fiber Input to the Medulla | 545 | ||
Physiological Role of the Peripheral Chemoreceptors in Cardiovascular Control | 545 | ||
Central Chemoreceptors | 545 | ||
Regulation of Cardiac Output | 545 | ||
Mechanisms intrinsic to the heart modulate both heart rate and stroke volume | 545 | ||
Intrinsic Control of Heart Rate | 545 | ||
Intrinsic Control of Stroke Volume | 545 | ||
Mechanisms extrinsic to the heart also modulate heart rate and stroke volume | 546 | ||
Baroreceptor Regulation | 546 | ||
Chemoreceptor Regulation | 546 | ||
Low-pressure baroreceptors in the atria respond to increased “fullness” of the vascular system, triggering tachycardia, renal vasodilation, and diuresis | 546 | ||
Atrial Receptors | 546 | ||
Ventricular Receptors | 547 | ||
Cardiac output is roughly proportional to effective circulating blood volume | 547 | ||
Matching of Venous Return and Cardiac Output | 548 | ||
Increases in cardiac output cause right atrial pressure to fall | 549 | ||
Changes in blood volume shift the vascular function curve to different RAPs, whereas changes in arteriolar tone alter the slope of the curve | 550 | ||
Because vascular function and cardiac function depend on each other, cardiac output and venous return match at exactly one value of RAP | 551 | ||
Intermediate- and Long-Term Control of the Circulation | 551 | ||
Endocrine and paracrine vasoactive compounds control the circulatory system on an intermediate- to long-term basis | 551 | ||
Biogenic Amines | 553 | ||
Peptides | 553 | ||
Prostaglandins | 554 | ||
Nitric Oxide | 554 | ||
Pathways for the renal control of ECF volume are the primary long-term regulators of mean arterial pressure | 554 | ||
References | 555 | ||
References | 555.e1 | ||
Books and Reviews | 555.e1 | ||
Journal Articles | 555.e1 | ||
24 Special Circulations | 556 | ||
The blood flow to individual organs must vary to meet the needs of the particular organ, as well as of the whole body | 556 | ||
Neural, myogenic, metabolic, and endothelial mechanisms control regional blood flow | 556 | ||
Neural Mechanisms | 556 | ||
Myogenic Mechanisms | 556 | ||
Metabolic Mechanisms | 556 | ||
Endothelial Mechanisms | 556 | ||
The Brain | 557 | ||
Anastomoses at the circle of Willis and among the branches of distributing arteries protect the blood supply to the brain, which is ~15% of resting cardiac output | 557 | ||
Arteries | 557 | ||
Veins | 557 | ||
Capillaries | 558 | ||
Lymphatics | 558 | ||
Vascular Volume | 558 | ||
Neural, metabolic, and myogenic mechanisms control blood flow to the brain | 558 | ||
Neural Control | 558 | ||
Metabolic Control | 559 | ||
Myogenic Control | 559 | ||
The neurovascular unit matches blood flow to local brain activity | 559 | ||
Autoregulation maintains a fairly constant cerebral blood flow across a broad range of perfusion pressures | 559 | ||
The Heart | 560 | ||
The coronary circulation receives 5% of the resting cardiac output from the left heart and mostly returns it to the right heart | 560 | ||
Extravascular compression impairs coronary blood flow during systole | 560 | ||
Myocardial blood flow parallels myocardial metabolism | 561 | ||
Although sympathetic stimulation directly constricts coronary vessels, accompanying metabolic effects predominate, producing an overall vasodilation | 562 | ||
Collateral vessel growth can provide blood flow to ischemic regions | 562 | ||
Vasodilator drugs may compromise myocardial flow through “coronary steal” | 562 | ||
The Skeletal Muscle | 562 | ||
A microvascular unit is the capillary bed supplied by a single terminal arteriole | 562 | ||
Metabolites released by active muscle trigger vasodilation and an increase in blood flow | 563 | ||
Sympathetic innervation increases the intrinsic tone of resistance vessels | 564 | ||
Rhythmic contraction promotes blood flow through the “muscle pump” | 565 | ||
The Splanchnic Organs | 565 | ||
The vascular supply to the gut is highly interconnected | 565 | ||
Blood flow to the gastrointestinal tract increases up to eight-fold after a meal (postprandial hyperemia) | 567 | ||
Sympathetic activity directly constricts splanchnic blood vessels, whereas parasympathetic activity indirectly dilates them | 567 | ||
Changes in the splanchnic circulation regulate total peripheral resistance and the distribution of blood volume | 568 | ||
Exercise and hemorrhage can substantially reduce splanchnic blood flow | 568 | ||
The liver receives its blood flow from both the systemic and the portal circulation | 568 | ||
The Skin | 569 | ||
The skin is the largest organ of the body | 569 | ||
Specialized arteriovenous anastomoses in apical skin help control heat loss | 570 | ||
Apical Skin | 570 | ||
Nonapical Skin | 571 | ||
Mechanical stimuli elicit local vascular responses in the skin | 571 | ||
White Reaction | 571 | ||
“Triple Response” | 571 | ||
References | 571 | ||
References | 571.e1 | ||
Books and Reviews | 571.e1 | ||
Journal Articles | 571.e1 | ||
25 Integrated Control of the Cardiovascular System | 572 | ||
Interaction among the Different Cardiovascular Control Systems | 572 | ||
The control of the cardiovascular system involves “linear,” “branched,” and “connected” interactions | 572 | ||
Regulation of the entire cardiovascular system depends on the integrated action of multiple subsystem controls as well as noncardiovascular controls | 572 | ||
Response to Erect Posture | 575 | ||
Because of gravity, standing up (orthostasis) tends to shift blood from the head and heart to veins in the legs | 575 | ||
The ANS mediates an “orthostatic response” that raises heart rate and peripheral vascular resistance and thus tends to restore mean arterial pressure | 576 | ||
Nonuniform Initial Distribution of Blood | 576 | ||
Nonuniform Distensibility of the Vessels | 576 | ||
Muscle Pumps | 576 | ||
Autonomic Reflexes | 576 | ||
Postural Hypotension | 576 | ||
Temperature Effects | 576 | ||
Responses to Acute Emotional Stress | 577 | ||
The fight-or-flight reaction is a sympathetic response that is centrally controlled in the cortex and hypothalamus | 577 | ||
The common faint reflects mainly a parasympathetic response caused by sudden emotional stress | 579 | ||
Response to Exercise | 580 | ||
Early physiologists suggested that muscle contraction leads to mechanical and chemical changes that trigger an increase in cardiac output | 580 | ||
Mechanical Response: Increased Venous Return | 580 | ||
Chemical Response: Local Vasodilation in Active Muscle | 580 | ||
Central command organizes an integrated cardiovascular response to exercise | 581 | ||
Muscle and baroreceptor reflexes, metabolites, venous return, histamine, epinephrine, and increased temperature reinforce the response to exercise | 581 | ||
Response to Hemorrhage | 583 | ||
After hemorrhage, cardiovascular reflexes restore mean arterial pressure | 583 | ||
Tachycardia and Increased Contractility | 585 | ||
Arteriolar Constriction | 585 | ||
Venous Constriction | 585 | ||
Circulating Vasoactive Agonists | 585 | ||
After hemorrhage, transcapillary refill, fluid conservation, and thirst restore the blood volume | 585 | ||
Transcapillary Refill | 585 | ||
Renal Conservation of Salt and Water | 586 | ||
Thirst | 586 | ||
Positive-feedback mechanisms cause irreversible hemorrhagic shock | 587 | ||
Failure of the Vasoconstrictor Response | 587 | ||
Failure of the Capillary Refill | 587 | ||
Failure of the Heart | 587 | ||
CNS Depression | 587 | ||
References | 587 | ||
References | 587.e1 | ||
Books and Reviews | 587.e1 | ||
Journal Articles | 587.e1 | ||
V The Respiratory System | 589 | ||
26 Organization of the Respiratory System | 590 | ||
Comparative Physiology of Respiration | 590 | ||
External respiration is the exchange of O2 and CO2 between the atmosphere and the mitochondria | 590 | ||
Diffusion is the major mechanism of external respiration for small aquatic organisms | 590 | ||
Convection enhances diffusion by producing steeper gradients across the diffusion barrier | 592 | ||
Surface area amplification enhances diffusion | 595 | ||
Respiratory pigments such as hemoglobin increase the carrying capacity of the blood for both O2 and CO2 | 595 | ||
Pathophysiology recapitulates phylogeny … in reverse | 595 | ||
Organization of the Respiratory System in Humans | 596 | ||
Humans optimize each aspect of external respiration—ventilation, circulation, area amplification, gas carriage, local control, and central control | 596 | ||
Conducting airways deliver fresh air to the alveolar spaces | 597 | ||
Alveolar air spaces are the site of gas exchange | 597 | ||
The lungs play important nonrespiratory roles, including filtering the blood, serving as a reservoir for the left ventricle, and performing several biochemical conversions | 600 | ||
Olfaction | 600 | ||
Processing of Inhaled Air Before It Reaches the Alveoli | 600 | ||
Left Ventricular Reservoir | 600 | ||
Filtering Small Emboli from the Blood | 600 | ||
Biochemical Reactions | 600 | ||
Lung Volumes and Capacities | 601 | ||
The spirometer measures changes in lung volume | 601 | ||
The volume of distribution of helium or nitrogen in the lung is an estimate of the RV | 602 | ||
Helium-Dilution Technique | 602 | ||
N2-Washout Method | 604 | ||
The plethysmograph, together with Boyle’s law, is a tool for estimation of RV | 604 | ||
References | 605 | ||
References | 605.e1 | ||
Books and Reviews | 605.e1 | ||
Journal Articles | 605.e1 | ||
27 Mechanics of Ventilation | 606 | ||
Static Properties of the Lung | 606 | ||
The balance between the outward elastic recoil of the chest wall and the inward elastic recoil of the lungs generates a subatmospheric intrapleural pressure | 606 | ||
Contraction of the diaphragm and selected intercostal muscles increases the volume of the thorax, producing an inspiration | 606 | ||
Relaxation of the muscles of inspiration produces a quiet expiration | 607 | ||
An increase of the static compliance makes it easier to inflate the lungs | 608 | ||
Surface tension at the air-water interface of the airways accounts for most of the elastic recoil of the lungs | 610 | ||
Pulmonary surfactant is a mixture of lipids—mainly dipalmitoylphosphatidylcholine—and apoproteins | 613 | ||
Pulmonary surfactant reduces surface tension and increases compliance | 615 | ||
Dynamic Properties of the Lung | 616 | ||
Airflow is proportional to the difference between alveolar and atmospheric pressure, but inversely proportional to airway resistance | 616 | ||
In the lung, airflow is transitional in most of the tracheobronchial tree | 617 | ||
The smallest airways contribute only slightly to total airway resistance in healthy lungs | 617 | ||
Vagal tone, histamine, and reduced lung volume all increase airway resistance | 620 | ||
Intrapleural pressure has a static component (−PTP) that determines lung volume and a dynamic component (Pa) that determines airflow | 620 | ||
Transpulmonary Pressure | 621 | ||
Alveolar Pressure | 621 | ||
During inspiration, a sustained negative shift in PIP causes Pa to become transiently more negative | 622 | ||
Dynamic compliance falls as respiratory frequency rises | 622 | ||
Transmural pressure differences cause airways to dilate during inspiration and to compress during expiration | 624 | ||
Static Conditions | 625 | ||
Inspiration | 625 | ||
Expiration | 626 | ||
Because of airway collapse, expiratory flow rates become independent of effort at low lung volumes | 626 | ||
References | 627 | ||
References | 627.e1 | ||
Books and Reviews | 627.e1 | ||
Journal Articles | 627.e1 | ||
28 Acid-Base Physiology | 628 | ||
pH and Buffers | 628 | ||
pH values vary enormously among different intracellular and extracellular compartments | 628 | ||
Buffers minimize the size of the pH changes produced by adding acid or alkali to a solution | 628 | ||
According to the Henderson-Hasselbalch equation, pH depends on the ratio [CO2]/[] | 629 | ||
has a far higher buffering power in an open than in a closed system | 630 | ||
Acid-Base Chemistry When Is the Only Buffer | 633 | ||
In the absence of other buffers, doubling causes pH to fall by 0.3 but causes almost no change in [] | 633 | ||
In the absence of other buffers, doubling [] causes pH to rise by 0.3 | 634 | ||
Acid-Base Chemistry in the Presence of and Buffers—The Davenport Diagram | 635 | ||
The Davenport diagram is a graphical tool for interpreting acid-base disturbances in blood | 635 | ||
The Buffer | 635 | ||
Buffers | 636 | ||
Solving the Problem | 637 | ||
The amount of formed or consumed during “respiratory” acid-base disturbances increases with | 637 | ||
Adding or removing an acid or base—at a constant —produces a “metabolic” acid-base disturbance | 638 | ||
During metabolic disturbances, makes a greater contribution to total buffering when pH and are high and when is low | 638 | ||
A metabolic change can compensate for a respiratory disturbance | 641 | ||
A respiratory change can compensate for a metabolic disturbance | 642 | ||
Position on a Davenport diagram defines the nature of an acid-base disturbance | 643 | ||
pH Regulation of Intracellular Fluid | 644 | ||
Ion transporters at the plasma membrane closely regulate the pH inside of cells | 644 | ||
Indirect interactions between K+ and H+ make it appear as if cells have a K-H exchanger | 645 | ||
Changes in intracellular pH are often a sign of changes in extracellular pH, and vice versa | 645 | ||
References | 646 | ||
References | 646.e1 | ||
Books and Reviews | 646.e1 | ||
Journal Articles | 646.e1 | ||
29 Transport of Oxygen and Carbon Dioxide in the Blood | 647 | ||
Carriage of O2 | 647 | ||
The amount of O2 dissolved in blood is far too small to meet the metabolic demands of the body | 647 | ||
Hemoglobin consists of two α and two β subunits, each of which has an iron-containing “heme” and a polypeptide “globin” | 647 | ||
The Hb-O2 dissociation curve has a sigmoidal shape because of cooperativity among the four subunits of the Hb molecule | 649 | ||
Increases in temperature, [CO2], and [H+], all of which are characteristic of metabolically active tissues, cause Hb to dump O2 | 652 | ||
Temperature | 652 | ||
Acid | 652 | ||
Carbon Dioxide | 653 | ||
2,3-Diphosphoglycerate reduces the affinity of adult, but not of fetal, Hb | 654 | ||
Carriage of CO2 | 655 | ||
Blood carries “total CO2” mainly as | 655 | ||
CO2 transport depends critically on carbonic anhydrase, the Cl-HCO3 exchanger, and Hb | 655 | ||
The high in the lungs causes the blood to dump CO2 | 657 | ||
The O2-CO2 diagram describes the interaction of and in the blood | 658 | ||
References | 659 | ||
References | 659.e1 | ||
Books and Reviews | 659.e1 | ||
Journal Articles | 659.e1 | ||
30 Gas Exchange in the Lungs | 660 | ||
Diffusion of Gases | 660 | ||
Gas flow across a barrier is proportional to diffusing capacity and concentration gradient (Fick’s law) | 660 | ||
The total flux of a gas between alveolar air and blood is the summation of multiple diffusion events along each pulmonary capillary during the respiratory cycle | 661 | ||
The flow of O2, CO, and CO2 between alveolar air and blood depends on the interaction of these gases with red blood cells | 663 | ||
Diffusion and Perfusion Limitations on Gas Transport | 664 | ||
The diffusing capacity normally limits the uptake of CO from alveolar air to blood | 664 | ||
Perfusion normally limits the uptake of N2O from alveolar air to blood | 666 | ||
In principle, CO transport could become perfusion limited and N2O transport could become diffusion limited under special conditions | 668 | ||
The uptake of CO provides an estimate of DL | 668 | ||
For both O2 and CO2, transport is normally perfusion limited | 671 | ||
Uptake of O2 | 671 | ||
Escape of CO2 | 673 | ||
Pathological changes that reduce DL do not necessarily produce hypoxia | 673 | ||
References | 674 | ||
References | 674.e1 | ||
Books and Reviews | 674.e1 | ||
Journal Articles | 674.e1 | ||
31 Ventilation and Perfusion of the Lungs | 675 | ||
Ventilation | 675 | ||
About 30% of total ventilation in a respiratory cycle is wasted ventilating anatomical dead space (i.e., conducting airways) | 675 | ||
The Fowler single-breath N2-washout technique estimates anatomical dead space | 676 | ||
The Bohr expired-[CO2] approach estimates physiological dead space | 677 | ||
Alveolar ventilation is the ratio of CO2 production rate to CO2 mole fraction in alveolar air | 679 | ||
Alveolar and arterial are inversely proportional to alveolar ventilation | 679 | ||
Alveolar and arterial rise with increased alveolar ventilation | 681 | ||
Because of the action of gravity on the lung, regional ventilation in an upright subject is normally greater at the base than the apex | 681 | ||
Restrictive and obstructive pulmonary diseases can exacerbate the nonuniformity of ventilation | 682 | ||
Restrictive Pulmonary Disease | 683 | ||
Obstructive Pulmonary Disease | 683 | ||
Perfusion of the Lung | 683 | ||
The pulmonary circulation has low pressure and resistance but high compliance | 683 | ||
Overall pulmonary vascular resistance is minimal at FRC | 684 | ||
Alveolar Vessels | 684 | ||
Extra-Alveolar Vessels | 685 | ||
Increases in pulmonary arterial pressure reduce pulmonary vascular resistance by recruiting and distending pulmonary capillaries | 685 | ||
Recruitment | 686 | ||
Distention | 687 | ||
Hypoxia is a strong vasoconstrictor, opposite to its effect in the systemic circulation | 687 | ||
Oxygen | 687 | ||
Carbon Dioxide and Low pH | 687 | ||
Autonomic Nervous System | 687 | ||
Hormones and Other Humoral Agents | 687 | ||
Because of gravity, regional perfusion in an upright subject is far greater near the base than the apex of the lung | 687 | ||
Zone 1: Pa > PPA > PPV | 689 | ||
Zone 2: PPA > Pa > PPV | 689 | ||
Zone 3: PPA > PPV > Pa | 689 | ||
Zone 4: PPA > PPV > Pa | 689 | ||
Matching Ventilation and Perfusion | 689 | ||
The greater the ventilation-perfusion ratio, the higher the and the lower the in the alveolar air | 689 | ||
Because of the action of gravity, the regional ratio in an upright subject is greater at the apex of the lung than at the base | 690 | ||
The ventilation of unperfused alveoli (local = ∞) triggers compensatory bronchoconstriction and a fall in surfactant production | 691 | ||
Alveolar Dead-Space Ventilation | 691 | ||
Redirection of Blood Flow | 692 | ||
Regulation of Local Ventilation | 692 | ||
The perfusion of unventilated alveoli (local = 0) triggers a compensatory hypoxic vasoconstriction | 692 | ||
Shunt | 692 | ||
Redirection of Airflow | 693 | ||
Asthma | 693 | ||
Normal Anatomical Shunts | 693 | ||
Pathological Shunts | 693 | ||
Regulation of Local Perfusion | 693 | ||
Even if whole-lung and are normal, exaggerated local mismatches produce hypoxia and respiratory acidosis | 693 | ||
Normal Lungs | 693 | ||
Alveolar Dead-Space Ventilation Affecting One Lung | 693 | ||
Shunt Affecting One Lung | 696 | ||
Mixed Mismatches | 699 | ||
References | 699 | ||
References | 699.e1 | ||
Books and Reviews | 699.e1 | ||
Journal Articles | 699.e1 | ||
32 Control of Ventilation | 700 | ||
Overview of the Respiratory Control System | 700 | ||
Automatic centers in the brainstem activate the respiratory muscles rhythmically and subconsciously | 700 | ||
Peripheral and central chemoreceptors—which sense , , and pH—drive the CPG | 700 | ||
Other receptors as well as higher brain centers also modulate ventilation | 702 | ||
Neurons That Control Ventilation | 702 | ||
The neurons that generate the respiratory rhythm are located in the medulla | 702 | ||
The pons modulates—but is not essential for—respiratory output | 702 | ||
The dorsal and ventral respiratory groups contain many neurons that fire in phase with respiratory motor output | 703 | ||
The dorsal respiratory group processes sensory input and contains primarily inspiratory neurons | 705 | ||
The ventral respiratory group is primarily motor and contains both inspiratory and expiratory neurons | 706 | ||
Generation of the Respiratory Rhythm | 706 | ||
Different RRNs fire at different times during inspiration and expiration | 706 | ||
The firing patterns of RRNs depend on the ion channels in their membranes and the synaptic inputs they receive | 706 | ||
Intrinsic Membrane Properties | 707 | ||
Synaptic Input | 707 | ||
Pacemaker properties and synaptic interactions may both contribute to the generation of the respiratory rhythm | 707 | ||
Pacemaker Activity | 707 | ||
Synaptic Interactions | 707 | ||
The respiratory CPG for eupnea could reside in a single site or in multiple sites, or could emerge from a complex network | 708 | ||
Restricted-Site Model | 708 | ||
Distributed Oscillator Models | 708 | ||
Emergent Property Model | 709 | ||
Chemical Control of Ventilation | 709 | ||
Peripheral Chemoreceptors | 710 | ||
Peripheral chemoreceptors (carotid and aortic bodies) respond to hypoxia, hypercapnia, and acidosis | 710 | ||
Sensitivity to Decreased Arterial | 710 | ||
Sensitivity to Increased Arterial | 710 | ||
Sensitivity to Decreased Arterial pH | 710 | ||
The glomus cell is the chemosensor in the carotid and aortic bodies | 710 | ||
Hypoxia, hypercapnia, and acidosis inhibit K+ channels, raise glomus cell [Ca2+]i, and release neurotransmitters | 712 | ||
Hypoxia N32-17 | 712 | ||
Hypercapnia | 712 | ||
Extracellular Acidosis | 713 | ||
Central Chemoreceptors | 713 | ||
The blood-brain barrier separates the central chemoreceptors in the medulla from arterial blood | 713 | ||
Central chemoreceptors are located in the ventrolateral medulla and other brainstem regions | 713 | ||
Some neurons of the medullary raphé and VLM are unusually pH sensitive | 714 | ||
Integrated Responses to Hypoxia, Hypercapnia, and Acidosis | 716 | ||
Hypoxia accentuates the acute response to respiratory acidosis | 716 | ||
Respiratory Acidosis | 716 | ||
Metabolic Acidosis | 716 | ||
Respiratory acidosis accentuates the acute response to hypoxia | 716 | ||
Modulation of Ventilatory Control | 717 | ||
Stretch and chemical/irritant receptors in the airways and lung parenchyma provide feedback about lung volume and the presence of irritants | 717 | ||
Slowly Adapting Pulmonary Stretch Receptors | 717 | ||
Rapidly Adapting Pulmonary Stretch (Irritant) Receptors | 717 | ||
C-Fiber Receptors | 717 | ||
Higher brain centers coordinate ventilation with other behaviors and can override the brainstem’s control of breathing | 718 | ||
Coordination with Voluntary Behaviors That Use Respiratory Muscles | 718 | ||
Coordination with Complex Nonventilatory Behaviors | 720 | ||
Modification by Affective States | 720 | ||
Balancing Conflicting Demands of Gas Exchange and Other Behaviors | 720 | ||
References | 720 | ||
References | 720.e1 | ||
Books and Reviews | 720.e1 | ||
Journal Articles | 720.e1 | ||
VI The Urinary System | 721 | ||
33 Organization of the Urinary System | 722 | ||
Functional Anatomy of the Kidney | 722 | ||
The kidneys are paired, retroperitoneal organs with vascular and epithelial elements | 722 | ||
The kidneys have a very high blood flow and glomerular capillaries flanked by afferent and efferent arterioles | 722 | ||
The functional unit of the kidney is the nephron | 723 | ||
The renal corpuscle has three components: vascular elements, the mesangium, and Bowman’s capsule and space | 724 | ||
The tubule components of the nephron include the proximal tubule, loop of Henle, distal tubule, and collecting duct | 727 | ||
The tightness of tubule epithelia increases from the proximal to the medullary collecting tubule | 729 | ||
Main Elements of Renal Function | 729 | ||
The nephron forms an ultrafiltrate of the blood plasma and then selectively reabsorbs the tubule fluid or secretes solutes into it | 729 | ||
The JGA is a region where each thick ascending limb contacts its glomerulus | 730 | ||
Sympathetic nerve fibers to the kidney regulate renal blood flow, glomerular filtration, and tubule reabsorption | 730 | ||
The kidneys, as endocrine organs, produce renin, 1,25-dihydroxyvitamin D, erythropoietin, prostaglandins, and bradykinin | 730 | ||
Measuring Renal Clearance and Transport | 730 | ||
The clearance of a solute is the virtual volume of plasma that would be totally cleared of a solute in a given time | 731 | ||
A solute’s urinary excretion is the algebraic sum of its filtered load, reabsorption by tubules, and secretion by tubules | 732 | ||
Microscopic techniques make it possible to measure single-nephron rates of filtration, absorption, and secretion | 733 | ||
Single-Nephron GFR | 733 | ||
Handling of Water by Tubule Segments in a Single Nephron | 733 | ||
Handling of Solutes by Tubule Segments in a Single Nephron | 734 | ||
The Ureters and Bladder | 735 | ||
The ureters propel urine from the renal pelvis to the bladder by peristaltic waves conducted along a syncytium of smooth-muscle cells | 735 | ||
Sympathetic, parasympathetic, and somatic fibers innervate the urinary bladder and its sphincters | 736 | ||
Bladder filling activates stretch receptors, initiating the micturition reflex, a spinal reflex under control of higher central nervous system centers | 738 | ||
References | 738 | ||
References | 738.e1 | ||
Books and Reviews | 738.e1 | ||
Journal Articles | 738.e1 | ||
34 Glomerular Filtration and Renal Blood Flow | 739 | ||
Glomerular Filtration | 739 | ||
A high glomerular filtration rate is essential for maintaining stable and optimal extracellular levels of solutes and water | 739 | ||
The clearance of inulin is a measure of GFR | 739 | ||
The clearance of creatinine is a useful clinical index of GFR | 741 | ||
Molecular size and electrical charge determine the filterability of solutes across the glomerular filtration barrier | 741 | ||
Hydrostatic pressure in glomerular capillaries favors glomerular ultrafiltration, whereas oncotic pressure in capillaries and hydrostatic pressure in Bowman’s space oppose it | 743 | ||
Renal Blood Flow | 745 | ||
Increased glomerular plasma flow leads to an increase in GFR | 745 | ||
Afferent and efferent arteriolar resistances control both glomerular plasma flow and GFR | 746 | ||
Peritubular capillaries provide tubules with nutrients and retrieve reabsorbed fluid | 747 | ||
Blood flow in the renal cortex exceeds that in the renal medulla | 749 | ||
The clearance of para-aminohippurate is a measure of RPF | 749 | ||
Control of Renal Blood Flow and Glomerular Filtration | 750 | ||
Autoregulation keeps RBF and GFR relatively constant | 750 | ||
Myogenic Response | 750 | ||
Tubuloglomerular Feedback | 750 | ||
Volume expansion and a high-protein diet increase GFR by reducing TGF | 751 | ||
Four factors that modulate RBF and GFR play key roles in regulating effective circulating volume | 752 | ||
Renin-Angiotensin-Aldosterone Axis | 752 | ||
Sympathetic Nerves | 752 | ||
Arginine Vasopressin | 752 | ||
Atrial Natriuretic Peptide | 752 | ||
Other vasoactive agents modulate RBF and GFR | 753 | ||
Epinephrine | 753 | ||
Dopamine | 753 | ||
Endothelins | 753 | ||
Prostaglandins | 753 | ||
Leukotrienes | 753 | ||
Nitric Oxide | 753 | ||
References | 753 | ||
References | 753.e2 | ||
Books and Reviews | 753.e2 | ||
Journal Articles | 753.e2 | ||
35 Transport of Sodium and Chloride | 754 | ||
Na+ and Cl− Transport by Different Segments of The Nephron | 754 | ||
Na+ and Cl− reabsorption decreases from proximal tubules to Henle’s loops to classic distal tubules to collecting tubules and ducts | 754 | ||
The tubule reabsorbs Na+ via both the transcellular and the paracellular pathways | 754 | ||
Transcellular Na+ Reabsorption | 754 | ||
Paracellular Na+ Reabsorption | 755 | ||
Na+ and Cl−, and Water Transport at the Cellular and Molecular Level | 756 | ||
Na+ reabsorption involves apical transporters or ENaCs and a basolateral Na-K pump | 756 | ||
Proximal Tubule | 756 | ||
Thin Limbs of Henle’s Loop | 757 | ||
Thick Ascending Limb | 757 | ||
Distal Convoluted Tubule | 758 | ||
Initial and Cortical Collecting Tubules | 758 | ||
Medullary Collecting Duct | 759 | ||
Cl− reabsorption involves both paracellular and transcellular pathways | 759 | ||
Proximal Tubule | 759 | ||
Thick Ascending Limb | 759 | ||
Distal Convoluted Tubule | 759 | ||
Collecting Ducts | 760 | ||
Water reabsorption is passive and secondary to solute transport | 761 | ||
Proximal Tubule | 761 | ||
Loop of Henle and Distal Nephron | 762 | ||
The kidney’s high O2 consumption reflects a high level of active Na+ transport | 762 | ||
Regulation of Na+ and Cl− Transport | 763 | ||
Glomerulotubular balance stabilizes fractional Na+ reabsorption by the proximal tubule in the face of changes in the filtered Na+ load | 763 | ||
The proximal tubule achieves GT balance by both peritubular and luminal mechanisms | 763 | ||
Peritubular Factors in the Proximal Tubule | 763 | ||
Luminal Factors in the Proximal Tubule | 765 | ||
ECF volume contraction or expansion upsets GT balance | 765 | ||
The distal nephron also increases Na+ reabsorption in response to an increased Na+ load | 765 | ||
Four parallel pathways that regulate effective circulating volume all modulate Na+ reabsorption | 765 | ||
Renin-Angiotensin-Aldosterone Axis | 765 | ||
Sympathetic Division of the Autonomic Nervous System | 766 | ||
Arginine Vasopressin (Antidiuretic Hormone) | 768 | ||
Atrial Natriuretic Peptide | 768 | ||
Dopamine, elevated plasma [Ca2+], an endogenous steroid, prostaglandins, and bradykinin all decrease Na+ reabsorption | 768 | ||
Dopamine | 768 | ||
Elevated Plasma [Ca2+] | 768 | ||
Endogenous Na-K Pump Inhibitor | 768 | ||
Prostaglandins | 769 | ||
Bradykinin | 769 | ||
References | 769 | ||
References | 769.e1 | ||
Books and Reviews | 769.e1 | ||
Journal Articles | 769.e1 | ||
36 Transport of Urea, Glucose, Phosphate, Calcium, Magnesium, and Organic Solutes | 770 | ||
Urea | 770 | ||
The kidney filters, reabsorbs, and secretes urea | 770 | ||
Urea excretion rises with increasing urinary flow | 772 | ||
Glucose | 772 | ||
The proximal tubule reabsorbs glucose via apical, electrogenic Na/glucose cotransport and basolateral facilitated diffusion | 772 | ||
Glucose excretion in the urine occurs only when the plasma concentration exceeds a threshold | 772 | ||
Other Organic Solutes | 773 | ||
The proximal tubule reabsorbs amino acids using a wide variety of apical and basolateral transporters | 773 | ||
An H+-driven cotransporter takes up oligopeptides across the apical membrane, whereas endocytosis takes up proteins and other large organic molecules | 776 | ||
Oligopeptides | 776 | ||
Proteins | 778 | ||
Two separate apical Na+-driven cotransporters reabsorb monocarboxylates and dicarboxylates/tricarboxylates | 779 | ||
The proximal tubule secretes PAH and a variety of other organic anions | 779 | ||
PAH secretion is an example of a Tm-limited mechanism | 781 | ||
The proximal tubule both reabsorbs and secretes urate | 781 | ||
Reabsorption | 783 | ||
Secretion | 783 | ||
The late proximal tubule secretes several organic cations | 783 | ||
Nonionic diffusion of neutral weak acids and bases across tubules explains why their excretion is pH dependent | 784 | ||
Phosphate | 785 | ||
The proximal tubule reabsorbs phosphate via apical Na/phosphate cotransporters | 785 | ||
Phosphate excretion in the urine already occurs at physiological plasma concentrations | 786 | ||
PTH inhibits apical Na/phosphate uptake, promoting phosphate excretion | 786 | ||
Fibroblast growth factor 23 and other phosphatonins also inhibit apical Na/phosphate uptake, promoting phosphate excretion | 787 | ||
Calcium | 787 | ||
Binding to plasma proteins and formation of Ca2+-anion complexes influence the filtration and reabsorption of Ca2 | 787 | ||
The proximal tubule reabsorbs two thirds of filtered Ca2+, with more distal segments reabsorbing nearly all of the remainder | 787 | ||
Proximal Tubule | 787 | ||
Thick Ascending Limb | 787 | ||
Distal Convoluted Tubule | 787 | ||
Transcellular Ca2+ movement is a two-step process, involving passive Ca2+ entry through apical channels and basolateral extrusion by electrogenic Na/Ca exchange and a Ca pump | 788 | ||
PTH and vitamin D stimulate—whereas high plasma Ca2+ inhibits—Ca2+ reabsorption | 789 | ||
Parathyroid Hormone | 789 | ||
Vitamin D | 789 | ||
Plasma Ca2+ Levels | 789 | ||
Diuretics | 789 | ||
Magnesium | 789 | ||
Most Mg2+ reabsorption takes place along the TAL | 789 | ||
Mg2+ reabsorption increases with depletion of Mg2+ or Ca2+, or with elevated PTH levels | 791 | ||
Mg2+ Depletion | 791 | ||
Hypermagnesemia and Hypercalcemia | 791 | ||
Hormones | 791 | ||
Diuretics | 791 | ||
References | 791 | ||
References | 791.e2 | ||
Books and Reviews | 791.e2 | ||
Journal Articles | 791.e2 | ||
37 Transport of Potassium | 792 | ||
Potassium Balance and the Overall Renal Handling of Potassium | 792 | ||
Changes in K+ concentrations can have major effects on cell and organ function | 792 | ||
K+ homeostasis involves external K+ balance between environment and body, and internal K+ balance between intracellular and extracellular compartments | 792 | ||
External K+ Balance | 792 | ||
Internal K+ Balance | 792 | ||
Ingested K+ moves transiently into cells for storage before excretion by the kidney | 792 | ||
The kidney excretes K+ by a combination of filtration, reabsorption, and secretion | 795 | ||
Potassium Transport by Different Segments of the Nephron | 795 | ||
The proximal tubule reabsorbs most of the filtered K+, whereas the distal nephron reabsorbs or secretes K+, depending on K+ intake | 795 | ||
Low Dietary K | 795 | ||
Normal or High Dietary K | 796 | ||
Medullary trapping of K+ helps to maximize K+ excretion when K+ intake is high | 796 | ||
Potassium Transport at the Cellular and Molecular Levels | 797 | ||
Passive K+ reabsorption along the proximal tubule follows Na+ and fluid movements | 797 | ||
K+ reabsorption along the TAL occurs predominantly via a transcellular route that exploits secondary active Na/K/Cl cotransport | 798 | ||
K+ secretion by principal and intercalated cells of the ICT and CCT involves active K+ uptake across the basolateral membrane | 799 | ||
K+ reabsorption by intercalated cells involves apical uptake via an H-K pump | 799 | ||
K+ reabsorption along the MCD is both passive and active | 799 | ||
Regulation of Renal Potassium Excretion | 799 | ||
Increased luminal flow increases K+ secretion | 799 | ||
An increased lumen-negative transepithelial potential increases K+ secretion | 800 | ||
Low luminal [Cl−] enhances K+ secretion | 800 | ||
Aldosterone increases K+ secretion | 800 | ||
Mineralocorticoids | 800 | ||
Glucocorticoids | 801 | ||
High K+ intake promotes renal K+ secretion | 801 | ||
Dietary K+ Loading | 801 | ||
Dietary K+ Deprivation | 803 | ||
Acidosis decreases K+ secretion | 803 | ||
Epinephrine reduces and AVP enhances K+ excretion | 803 | ||
Opposing factors stabilize K+ secretion | 803 | ||
Attenuating Effects | 804 | ||
Additive Effects | 804 | ||
References | 805 | ||
References | 805.e2 | ||
Books and Reviews | 805.e2 | ||
Journal Articles | 805.e2 | ||
38 Urine Concentration and Dilution | 806 | ||
Water Balance and the Overall Renal Handling of Water | 806 | ||
The kidney can generate a urine as dilute as 40 mOsm (one seventh of plasma osmolality) or as concentrated as 1200 mOsm (four times plasma osmolality) | 806 | ||
Free-water clearance () is positive if the kidney produces urine that is less concentrated than plasma and negative if the kidney produces urine that is more concentrated than plasma | 806 | ||
Isosmotic Urine | 807 | ||
Dilute Urine | 807 | ||
Concentrated Urine | 807 | ||
Water Transport by Different Segments of the Nephron | 807 | ||
The kidney concentrates urine by driving water via osmosis from the tubule lumen into a hyperosmotic interstitium | 807 | ||
Tubule fluid is isosmotic in the proximal tubule, becomes dilute in the loop of Henle, and then either remains dilute or becomes concentrated by the end of the collecting duct | 808 | ||
Generation of a Hyperosmotic Medulla and Urine | 809 | ||
The renal medulla is hyperosmotic to blood plasma during both antidiuresis (low urine flow) and water diuresis | 809 | ||
NaCl transport generates only a ~200-mOsm gradient across any portion of the ascending limb, but countercurrent exchange can multiply this single effect to produce a 900-mOsm gradient between cortex and papilla | 809 | ||
The single effect is the result of passive NaCl reabsorption in the thin ascending limb and active NaCl reabsorption in the TAL | 811 | ||
The IMCD reabsorbs urea, producing high levels of urea in the interstitium of the inner medulla | 811 | ||
Urea Handling | 811 | ||
Urea Recycling | 813 | ||
The vasa recta’s countercurrent exchange and relatively low blood flow minimize washout of medullary hyperosmolality | 813 | ||
The MCD produces a concentrated urine by osmosis, driven by the osmotic gradient between the medullary interstitium and the lumen | 816 | ||
Regulation by Arginine Vasopressin | 817 | ||
AVP increases water permeability in all nephron segments beyond the DCT | 817 | ||
AVP, via cAMP, causes vesicles containing AQP2 to fuse with apical membranes of principal cells of collecting tubules and ducts | 818 | ||
AVP increases NaCl reabsorption in the outer medulla and urea reabsorption in the IMCD, enhancing urinary concentrating ability | 818 | ||
References | 820 | ||
References | 820.e2 | ||
Books and Reviews | 820.e2 | ||
Journal Articles | 820.e2 | ||
39 Transport of Acids and Bases | 821 | ||
Acid-Base Balance and the Overall Renal Handling of Acid | 821 | ||
Whereas the lungs excrete the large amount of CO2 formed by metabolism, the kidneys are crucial for excreting nonvolatile acids | 821 | ||
To maintain acid-base balance, the kidney must not only reabsorb virtually all filtered but also secrete generated nonvolatile acids | 821 | ||
Secreted H+ titrates to CO2 ( reabsorption) and also titrates filtered buffers and endogenously produced NH3 | 823 | ||
Titration of Filtered (“ Reabsorption”) | 823 | ||
Titration of Filtered Buffers (Titratable-Acid Formation) | 824 | ||
Titration of Filtered and Secreted NH3 (Ammonium Excretion) | 825 | ||
Acid-Base Transport by Different Segments of the Nephron | 825 | ||
The nephron reclaims virtually all the filtered in the proximal tubule (~80%), thick ascending limb (~10%), and distal nephron (~10%) | 825 | ||
The nephron generates new , mostly in the proximal tubule | 825 | ||
Formation of Titratable Acid | 825 | ||
Excretion | 826 | ||
Acid-Base Transport at the Cellular and Molecular Levels | 827 | ||
H+ moves across the apical membrane from tubule cell to lumen by Na-H exchange, electrogenic H pumping, and K-H pumping | 827 | ||
Na-H Exchanger | 827 | ||
Electrogenic H Pump | 827 | ||
H-K Exchange Pump | 827 | ||
CAs in the lumen and cytosol stimulate H+ secretion by accelerating the interconversion of CO2 and | 828 | ||
Apical CA (CA IV) | 828 | ||
Cytoplasmic CA (CA II) | 828 | ||
Basolateral CA (CA IV and CA XII) | 828 | ||
Inhibition of CA | 829 | ||
efflux across the basolateral membrane takes place by electrogenic Na/HCO3 cotransport and Cl-HCO3 exchange | 829 | ||
Electrogenic Na/HCO3 Cotransport | 829 | ||
Cl-HCO3 Exchange | 829 | ||
is synthesized by proximal tubules, partly reabsorbed in the loop of Henle, and secreted passively into papillary collecting ducts | 829 | ||
Regulation of Renal Acid Secretion | 832 | ||
Respiratory acidosis stimulates renal H+ secretion | 832 | ||
Metabolic acidosis stimulates both proximal H+ secretion and NH3 production | 833 | ||
Metabolic alkalosis reduces proximal H+ secretion and, in the CCT, may even provoke secretion | 833 | ||
A rise in GFR increases delivery to the tubules, enhancing reabsorption (glomerulotubular balance for ) | 834 | ||
Extracellular volume contraction—via ANG II, aldosterone, and sympathetic activity—stimulates renal H+ secretion | 834 | ||
Hypokalemia increases renal H+ secretion | 834 | ||
Both glucocorticoids and mineralocorticoids stimulate acid secretion | 835 | ||
Diuretics can change H+ secretion, depending on how they affect transepithelial voltage, ECF volume, and plasma [K+] | 835 | ||
References | 835 | ||
References | 835.e2 | ||
Books and Reviews | 835.e2 | ||
Journal Articles | 835.e2 | ||
40 Integration of Salt and Water Balance | 836 | ||
Sodium Balance | 836 | ||
Water Balance | 836 | ||
Control of Extracellular Fluid Volume | 836 | ||
In the steady state, Na+ intake via the gastrointestinal tract equals Na+ output from renal and extrarenal pathways | 836 | ||
The kidneys increase Na+ excretion in response to an increase in ECF volume, not to an increase in extracellular Na+ concentration | 837 | ||
It is not the ECF volume as a whole, but the effective circulating volume, that regulates Na+ excretion | 838 | ||
Decreases in effective circulating volume trigger four parallel effector pathways to decrease renal Na+ excretion | 838 | ||
Increased activity of the renin-angiotensin-aldosterone axis is the first of four parallel pathways that correct a low effective circulating volume | 841 | ||
Increased sympathetic nerve activity, increased AVP, and decreased ANP are the other three parallel pathways that correct a low effective circulating volume | 842 | ||
Renal Sympathetic Nerve Activity | 842 | ||
Arginine Vasopressin (Antidiuretic Hormone) | 843 | ||
Atrial Natriuretic Peptide | 843 | ||
High arterial pressure raises Na+ excretion by hemodynamic mechanisms, independent of changes in effective circulating volume | 843 | ||
Large and Acute Decrease in Arterial Blood Pressure | 843 | ||
Large Increase in Arterial Pressure | 843 | ||
Control of Water Content (Extracellular Osmolality) | 844 | ||
Increased plasma osmolality stimulates hypothalamic osmoreceptors that trigger the release of AVP, inhibiting water excretion | 844 | ||
Hypothalamic neurons synthesize AVP and transport it along their axons to the posterior pituitary, where they store it in nerve terminals prior to release | 844 | ||
Increased osmolality stimulates a second group of osmoreceptors that trigger thirst, which promotes water intake | 845 | ||
Several nonosmotic stimuli also enhance AVP secretion | 846 | ||
Reduced Effective Circulating Volume | 846 | ||
Volume Expansion | 847 | ||
Pregnancy | 847 | ||
Other Factors | 848 | ||
Decreased effective circulating volume and low arterial pressure also trigger thirst | 849 | ||
Defense of the effective circulating volume usually has priority over defense of osmolality | 849 | ||
References | 849 | ||
References | 849.e2 | ||
Books and Reviews | 849.e2 | ||
Journal Articles | 849.e2 | ||
VII The Gastrointestinal System | 851 | ||
41 Organization of the Gastrointestinal System | 852 | ||
Overview of Digestive Processes | 852 | ||
The gastrointestinal tract is a tube that is specialized along its length for the sequential processing of food | 852 | ||
Assimilation of dietary food substances requires digestion as well as absorption | 852 | ||
Digestion requires enzymes secreted in the mouth, stomach, pancreas, and small intestine | 853 | ||
Ingestion of food initiates multiple endocrine, neural, and paracrine responses | 853 | ||
In addition to its function in nutrition, the GI tract plays important roles in excretion, fluid and electrolyte balance, and immunity | 855 | ||
Regulation of Gastrointestinal Function | 855 | ||
The ENS is a “minibrain” with sensory neurons, interneurons, and motor neurons | 855 | ||
ACh, peptides, and bioactive amines are the ENS neurotransmitters that regulate epithelial and motor function | 856 | ||
The brain-gut axis is a bidirectional system that controls GI function via the ANS, GI hormones, and the immune system | 856 | ||
Gastrointestinal Motility | 858 | ||
Tonic and rhythmic contractions of smooth muscle are responsible for churning, peristalsis, and reservoir action | 858 | ||
Segments of the GI tract have both longitudinal and circular arrays of muscles and are separated by sphincters that consist of specialized circular muscles | 858 | ||
Location of a sphincter determines its function | 859 | ||
Upper Esophageal Sphincter | 859 | ||
Lower Esophageal Sphincter | 859 | ||
Pyloric Sphincter | 860 | ||
Ileocecal Sphincter | 860 | ||
Internal and External Anal Sphincters | 860 | ||
Motility of the small intestine achieves both churning and propulsive movement, and its temporal pattern differs in the fed and fasted states | 860 | ||
Motility of the large intestine achieves both propulsive movement and a reservoir function | 862 | ||
References | 862 | ||
References | 862.e2 | ||
Books and Reviews | 862.e2 | ||
Journal Articles | 862.e2 | ||
42 Gastric Function | 863 | ||
Functional Anatomy of the Stomach | 863 | ||
The mucosa is composed of surface epithelial cells and glands | 863 | ||
With increasing rates of secretion of gastric juice, the H+ concentration rises and the Na+ concentration falls | 863 | ||
The proximal portion of the stomach secretes acid, pepsinogens, intrinsic factor, bicarbonate, and mucus, whereas the distal part releases gastrin and somatostatin | 863 | ||
Corpus | 863 | ||
Antrum | 865 | ||
The stomach accommodates food, mixes it with gastric secretions, grinds it, and empties the chyme into the duodenum | 865 | ||
Acid Secretion | 865 | ||
The parietal cell has a specialized tubulovesicular structure that increases apical membrane area when the cell is stimulated to secrete acid | 865 | ||
An H-K pump is responsible for gastric acid secretion by parietal cells | 865 | ||
Three secretagogues (acetylcholine, gastrin, and histamine) directly and indirectly induce acid secretion by parietal cells | 866 | ||
The three acid secretagogues act through either Ca2+/diacylglycerol or cAMP | 867 | ||
Antral and duodenal G cells release gastrin, whereas ECL cells in the corpus release histamine | 867 | ||
Gastric D cells release somatostatin, the central inhibitor of acid secretion | 868 | ||
Several enteric hormones (“enterogastrone”) and prostaglandins inhibit gastric acid secretion | 870 | ||
A meal triggers three phases of acid secretion | 870 | ||
Basal State | 870 | ||
Cephalic Phase | 871 | ||
Gastric Phase | 871 | ||
Intestinal Phase | 872 | ||
Pepsinogen Secretion | 872 | ||
Chief cells, triggered by both cAMP and Ca2+ pathways, secrete multiple pepsinogens that initiate protein digestion | 872 | ||
Agonists Acting via cAMP | 873 | ||
Agonists Acting via Ca2 | 873 | ||
Low pH is required for both pepsinogen activation and pepsin activity | 873 | ||
Protection of the Gastric Surface Epithelium and Neutralization of Acid in the Duodenum | 874 | ||
Vagal stimulation and irritation stimulate gastric mucous cells to secrete mucins | 874 | ||
Gastric surface cells secrete , stimulated by acetylcholine, acids, and prostaglandins | 874 | ||
Mucus protects the gastric surface epithelium by trapping an -rich fluid near the apical border of these cells | 874 | ||
Acid entry into the duodenum induces S cells to release secretin, triggering the pancreas and duodenum to secrete | 875 | ||
Filling and Emptying of the Stomach | 876 | ||
Gastric motor activity plays a role in filling, churning, and emptying | 876 | ||
Filling of the stomach is facilitated by both receptive relaxation and gastric accommodation | 877 | ||
The stomach churns its contents until the particles are small enough to be gradually emptied into the duodenum | 877 | ||
References | 878 | ||
References | 878.e1 | ||
Books and Reviews | 878.e1 | ||
Journal Articles | 878.e1 | ||
43 Pancreatic and Salivary Glands | 879 | ||
Overview of Exocrine Gland Physiology | 879 | ||
The pancreas and major salivary glands are compound exocrine glands | 879 | ||
Acinar cells are specialized protein-synthesizing cells | 879 | ||
Duct cells are epithelial cells specialized for fluid and electrolyte transport | 881 | ||
Goblet cells contribute to mucin production in exocrine glands | 881 | ||
Pancreatic Acinar Cell | 882 | ||
The acinar cell secretes digestive proteins in response to stimulation | 882 | ||
Acetylcholine and cholecystokinin mediate the regulated secretion of proteins by pancreatic acinar cells | 882 | ||
Ca2+ is the major second messenger for the secretion of proteins by pancreatic acinar cells | 883 | ||
Ca2 | 883 | ||
cAMP | 883 | ||
Effectors | 884 | ||
In addition to proteins, the pancreatic acinar cell secretes a plasma-like fluid | 884 | ||
Pancreatic Duct Cell | 885 | ||
The pancreatic duct cell secretes isotonic NaHCO3 | 885 | ||
Secretin (via cAMP) and ACh (via Ca2+) stimulate secretion by pancreatic ducts | 886 | ||
Apical membrane chloride channels are important sites of neurohumoral regulation | 887 | ||
Pancreatic duct cells may also secrete glycoproteins | 887 | ||
Composition, Function, and Control of Pancreatic Secretion | 887 | ||
Pancreatic juice is a protein-rich, alkaline secretion | 887 | ||
In the fasting state, levels of secreted pancreatic enzymes oscillate at low levels | 888 | ||
CCK from duodenal I cells stimulates acinar enzyme secretion, and secretin from S cells stimulates and fluid secretion by ducts | 889 | ||
A meal triggers cephalic, gastric, and intestinal phases of pancreatic secretion | 890 | ||
Cephalic Phase | 890 | ||
Gastric Phase | 890 | ||
Intestinal Phase | 890 | ||
The pancreas has large reserves of digestive enzymes for carbohydrates and proteins, but not for lipids | 892 | ||
Fat in the distal part of the small intestine inhibits pancreatic secretion | 892 | ||
Several mechanisms protect the pancreas from autodigestion | 892 | ||
Salivary Acinar Cell | 893 | ||
Different salivary acinar cells secrete different proteins | 893 | ||
Cholinergic and adrenergic neural pathways are the most important physiological activators of regulated secretion by salivary acinar cells | 894 | ||
Both cAMP and Ca2+ mediate salivary acinar secretion | 894 | ||
Salivary Duct Cell | 894 | ||
Salivary duct cells produce a hypotonic fluid that is poor in NaCl and rich in KHCO3 | 894 | ||
Parasympathetic stimulation decreases Na+ absorption, whereas aldosterone increases Na+ absorption by duct cells | 895 | ||
Salivary duct cells also secrete and take up proteins | 895 | ||
Composition, Function, and Control of Salivary Secretion | 896 | ||
Depending on protein composition, salivary secretions can be serous, seromucous, or mucous | 896 | ||
At low flow rates, the saliva is hypotonic and rich in K+, whereas at higher flow rates, its composition approaches that of plasma | 896 | ||
Parasympathetic stimulation increases salivary secretion | 897 | ||
Parasympathetic Control | 897 | ||
Sympathetic Control | 897 | ||
References | 898 | ||
References | 898.e1 | ||
Books and Reviews | 898.e1 | ||
Journal Articles | 898.e1 | ||
44 Intestinal Fluid and Electrolyte Movement | 899 | ||
Functional Anatomy | 899 | ||
Both the small and large intestine absorb and secrete fluid and electrolytes, whereas only the small intestine absorbs nutrients | 899 | ||
The small intestine has a villus-crypt organization, whereas the colon has surface epithelial cells with interspersed crypts | 899 | ||
The surface area of the small intestine is amplified by folds, villi, and microvilli; amplification is less marked in the colon | 901 | ||
Overview of Fluid and Electrolyte Movement in the Intestines | 901 | ||
The small intestine absorbs ~6.5 L/day of an ~8.5-L fluid load that is presented to it, and the colon absorbs ~1.9 L/day | 901 | ||
The small intestine absorbs net amounts of water, Na+, Cl−, and K+ and secretes , whereas the colon absorbs net amounts of water, Na+, and Cl− and secretes both K+ and | 901 | ||
The intestines absorb and secrete solutes by both active and passive mechanisms | 902 | ||
Intestinal fluid movement is always coupled to solute movement, and sometimes solute movement is coupled to fluid movement by solvent drag | 903 | ||
The resistance of the tight junctions primarily determines the transepithelial resistance of intestinal epithelia | 903 | ||
Cellular Mechanisms of Na+ Absorption | 903 | ||
Na/glucose and Na/amino-acid cotransport in the small intestine is a major mechanism for postprandial Na+ absorption | 903 | ||
Electroneutral Na-H exchange in the duodenum and jejunum is responsible for Na+ absorption that is stimulated by luminal alkalinity | 904 | ||
Parallel Na-H and Cl-HCO3 exchange in the ileum and proximal part of the colon is the primary mechanism of Na+ absorption during the interdigestive period | 905 | ||
Epithelial Na+ channels are the primary mechanism of “electrogenic” Na+ absorption in the distal part of the colon | 905 | ||
Cellular Mechanisms of Cl− Absorption and Secretion | 905 | ||
Voltage-dependent Cl− absorption represents coupling of Cl− absorption to electrogenic Na+ absorption in both the small intestine and the large intestine | 906 | ||
Electroneutral Cl-HCO3 exchange results in Cl− absorption and secretion in the ileum and colon | 906 | ||
Parallel Na-H and Cl-HCO3 exchange in the ileum and the proximal part of the colon mediates Cl− absorption during the interdigestive period | 907 | ||
Electrogenic Cl− secretion occurs in crypts of both the small and the large intestine | 907 | ||
Cellular Mechanisms of K+ Absorption and Secretion | 908 | ||
Overall net transepithelial K+ movement is absorptive in the small intestine and secretory in the colon | 908 | ||
K+ absorption in the small intestine probably occurs via solvent drag | 908 | ||
Passive K+ secretion is the primary mechanism for net colonic secretion | 908 | ||
Active K+ secretion is also present throughout the large intestine and is induced both by aldosterone and by cAMP | 909 | ||
Aldosterone | 909 | ||
cAMP and Ca2 | 909 | ||
Active K+ absorption takes place only in the distal portion of the colon and is energized by an apical H-K pump | 910 | ||
Regulation of Intestinal Ion Transport | 910 | ||
Chemical mediators from the enteric nervous system, endocrine cells, and immune cells in the lamina propria may be either secretagogues or absorptagogues | 910 | ||
Secretagogues can be classified by their type and by the intracellular second-messenger system that they stimulate | 910 | ||
Mineralocorticoids, glucocorticoids, and somatostatin are absorptagogues | 912 | ||
References | 913 | ||
References | 913.e1 | ||
Books and Reviews | 913.e1 | ||
Journal Articles | 913.e1 | ||
45 Nutrient Digestion and Absorption | 914 | ||
Carbohydrate Digestion | 914 | ||
Carbohydrates, providing ~45% of total energy needs of Western diets, require hydrolysis to monosaccharides before absorption | 914 | ||
Luminal digestion begins with the action of salivary amylase and finishes with pancreatic amylase | 916 | ||
“Membrane digestion” involves hydrolysis of oligosaccharides to monosaccharides by brush-border disaccharidases | 916 | ||
Carbohydrate Absorption | 919 | ||
SGLT1 is responsible for the Na+-coupled uptake of glucose and galactose across the apical membrane | 919 | ||
The GLUT transporters mediate the facilitated diffusion of fructose at the apical membrane and of all three monosaccharides at the basolateral membrane | 919 | ||
Protein Digestion | 920 | ||
Proteins require hydrolysis to oligopeptides or amino acids before absorption in the small intestine | 920 | ||
Luminal digestion of protein involves both gastric and pancreatic proteases, and yields amino acids and oligopeptides | 921 | ||
Brush-border peptidases fully digest some oligopeptides to amino acids, whereas cytosolic peptidases digest oligopeptides that directly enter the enterocyte | 922 | ||
Protein, Peptide, and Amino-Acid Absorption | 922 | ||
Absorption of whole protein by apical endocytosis occurs primarily during the neonatal period | 922 | ||
The apical absorption of dipeptides, tripeptides, and tetrapeptides occurs via an H+-driven cotransporter | 922 | ||
Amino acids enter enterocytes via one or more group-specific apical transporters | 923 | ||
At the basolateral membrane, amino acids exit enterocytes via Na+-independent transporters and enter via Na+-dependent transporters | 923 | ||
Lipid Digestion | 925 | ||
Natural lipids of biological origin are sparingly soluble in water | 925 | ||
Dietary lipids are predominantly TAGs | 925 | ||
Endogenous lipids are phospholipids and cholesterol from bile and membrane lipids from desquamated intestinal epithelial cells | 927 | ||
The mechanical disruption of dietary lipids in the mouth and stomach produces an emulsion of lipid particles | 927 | ||
Lingual and gastric (acid) lipase initiate lipid digestion | 927 | ||
Pancreatic (alkaline) lipase, colipase, milk lipase, and other esterases—aided by bile salts—complete lipid hydrolysis in the duodenum and jejunum | 928 | ||
Lipid Absorption | 929 | ||
Products of lipolysis enter the bulk water phase of the intestinal lumen as vesicles, mixed micelles, and monomers | 929 | ||
Lipids diffuse as mixed micelles and monomers through unstirred layers before crossing the jejunal enterocyte brush border | 930 | ||
The enterocyte re-esterifies lipid components and assembles them into chylomicrons | 930 | ||
The enterocyte secretes chylomicrons into the lymphatics during feeding and secretes VLDLs during fasting | 932 | ||
Digestion and Absorption of Vitamins and Minerals | 933 | ||
Intestinal absorption of fat-soluble vitamins follows the pathways of lipid absorption and transport | 933 | ||
Dietary folate (PteGlu7) must be deconjugated by a brush-border enzyme before absorption by an anion exchanger at the apical membrane | 933 | ||
Vitamin B12 (cobalamin) binds to haptocorrin in the stomach and then to intrinsic factor in the small intestine before endocytosis by enterocytes in the ileum | 935 | ||
Ca2+ absorption, regulated primarily by vitamin D, occurs by active transport in the duodenum and by diffusion throughout the small intestine | 938 | ||
Mg2+ absorption occurs by an active process in the ileum | 939 | ||
Heme and nonheme iron are absorbed in the duodenum by distinct cellular mechanisms | 939 | ||
Nonheme Iron | 939 | ||
Heme Iron | 941 | ||
Nutritional Requirements | 941 | ||
No absolute daily requirement for carbohydrate or fat intake exists | 941 | ||
The daily protein requirement for adult humans is typically 0.8 g/kg body weight but is higher in pregnant women, postsurgical patients, and athletes | 941 | ||
Minerals and vitamins are not energy sources but are necessary for certain enzymatic reactions, for protein complexes, or as precursors for biomolecules | 942 | ||
Minerals | 942 | ||
Vitamins | 942 | ||
Excessive intake of vitamins and minerals has mixed effects on bodily function | 943 | ||
References | 943 | ||
References | 943.e1 | ||
Books and Reviews | 943.e1 | ||
Journal Articles | 943.e1 | ||
46 Hepatobiliary Function | 944 | ||
Overview of Liver Physiology | 944 | ||
The liver biotransforms and degrades substances taken up from blood and either returns them to the circulation or excretes them into bile | 944 | ||
The liver stores carbohydrates, lipids, vitamins, and minerals; it synthesizes carbohydrates, protein, and intermediary metabolites | 944 | ||
Functional Anatomy of the Liver and Biliary Tree | 944 | ||
Hepatocytes are secretory epithelial cells separating the lumen of bile canaliculi from the fenestrated endothelium of sinusoids | 944 | ||
The liver contains endothelial cells, macrophages (Kupffer cells), and stellate cells (Ito cells) within the sinusoidal spaces | 946 | ||
The liver has a dual blood supply, but a single venous drainage system | 946 | ||
Hepatocytes can be thought of as being arranged as classic hepatic lobules, portal lobules, or acinar units | 946 | ||
Periportal hepatocytes specialize in oxidative metabolism, whereas pericentral hepatocytes detoxify drugs | 946 | ||
Bile drains from canaliculi into small terminal ductules, then into larger ducts, and eventually, via a single common duct, into the duodenum | 948 | ||
Uptake, Processing, and Secretion of Compounds by Hepatocytes | 949 | ||
An Na-K pump at the basolateral membranes of hepatocytes provides the energy for transporting a wide variety of solutes via channels and transporters | 951 | ||
Hepatocytes take up bile acids, other organic anions, and organic cations across their basolateral (sinusoidal) membranes | 951 | ||
Bile Acids and Salts | 951 | ||
Organic Anions | 951 | ||
Bilirubin | 951 | ||
Organic Cations | 953 | ||
Neutral Organic Compounds | 954 | ||
Inside the hepatocyte, the basolateral-to-apical movement of many compounds occurs by protein-bound or vesicular routes | 954 | ||
Bile Salts | 954 | ||
Bilirubin | 954 | ||
In phase I of the biotransformation of organic anions and other compounds, hepatocytes use mainly cytochrome P-450 enzymes | 954 | ||
In phase II of biotransformation, conjugation of phase I products makes them more water soluble for secretion into blood or bile | 955 | ||
In phase III of biotransformation, hepatocytes excrete products of phase I and II into bile or sinusoidal blood | 956 | ||
The interactions of xenobiotics with nuclear receptors control phase I, II, and III | 956 | ||
Hepatocytes secrete bile acids, organic anions, organic cations, and lipids across their apical (canalicular) membranes | 957 | ||
Bile Salts | 957 | ||
Organic Anions | 957 | ||
Organic Cations | 957 | ||
Biliary Lipids | 957 | ||
Hepatocytes take up proteins across their basolateral membranes by receptor-mediated endocytosis and fluid-phase endocytosis | 957 | ||
Bile Formation | 958 | ||
The secretion of canalicular bile is active and isotonic | 958 | ||
Major organic molecules in bile include bile acids, cholesterol, and phospholipids | 958 | ||
Canalicular bile flow has a constant component driven by the secretion of small organic molecules and a variable component driven by the secretion of bile acids | 959 | ||
Bile Acid–Independent Flow in the Canaliculi | 960 | ||
Bile Acid–Dependent Flow in the Canaliculi | 960 | ||
Secretin stimulates the cholangiocytes of ductules and ducts to secrete a watery, -rich fluid | 960 | ||
The gallbladder stores bile and delivers it to the duodenum during a meal | 961 | ||
The relative tones of the gallbladder and sphincter of Oddi determine whether bile flows from the common hepatic duct into the gallbladder or into the duodenum | 961 | ||
Enterohepatic Circulation of Bile Acids | 962 | ||
The enterohepatic circulation of bile acids is a loop consisting of secretion by the liver, reabsorption by the intestine, and return to the liver in portal blood for repeat secretion into bile | 962 | ||
Efficient intestinal conservation of bile acids depends on active apical absorption in the terminal ileum and passive absorption throughout the intestinal tract | 962 | ||
The Liver as a Metabolic Organ | 964 | ||
The liver can serve as either a source or a sink for glucose | 964 | ||
The liver synthesizes a variety of important plasma proteins (e.g., albumin, coagulation factors, and carriage proteins) and metabolizes dietary amino acids | 965 | ||
Protein Synthesis | 965 | ||
Amino-Acid Uptake | 965 | ||
Amino-Acid Metabolism | 965 | ||
The liver obtains dietary triacylglycerols and cholesterol by taking up remnant chylomicrons via receptor-mediated endocytosis | 966 | ||
Cholesterol, synthesized primarily in the liver, is an important component of cell membranes and serves as a precursor for bile acids and steroid hormones | 968 | ||
Synthesis of Cholesterol | 968 | ||
The liver is the central organ for cholesterol homeostasis and for the synthesis and degradation of LDL | 969 | ||
The liver is the prime site for metabolism and storage of the fat-soluble vitamins A, D, E, and K | 970 | ||
Vitamin A | 970 | ||
Vitamin D | 970 | ||
Vitamin E | 970 | ||
Vitamin K | 970 | ||
The liver stores copper and iron | 970 | ||
Copper | 970 | ||
Iron | 971 | ||
References | 971 | ||
References | 971.e1 | ||
Books and Reviews | 971.e1 | ||
Journal Articles | 971.e1 | ||
VIII The Endocrine System | 973 | ||
47 Organization of Endocrine Control | 974 | ||
Principles of Endocrine Function | 974 | ||
Chemical signaling can occur through endocrine, paracrine, or autocrine pathways | 974 | ||
Endocrine Glands | 974 | ||
Paracrine Factors | 974 | ||
Hormones may be peptides, metabolites of single amino acids, or metabolites of cholesterol | 975 | ||
Hormones can circulate either free or bound to carrier proteins | 976 | ||
Immunoassays allow measurement of circulating hormones | 976 | ||
Hormones can have complementary and antagonistic actions | 976 | ||
Endocrine regulation occurs through feedback control | 977 | ||
Endocrine regulation can involve hierarchic levels of control | 978 | ||
The anterior pituitary regulates reproduction, growth, energy metabolism, and stress responses | 978 | ||
The posterior pituitary regulates water balance and uterine contraction | 979 | ||
Peptide Hormones | 981 | ||
Specialized endocrine cells synthesize, store, and secrete peptide hormones | 981 | ||
Peptide hormones bind to cell-surface receptors and activate a variety of signal-transduction systems | 981 | ||
G Proteins Coupled to Adenylyl Cyclase | 982 | ||
G Proteins Coupled to Phospholipase C | 982 | ||
G Proteins Coupled to Phospholipase A2 | 984 | ||
Guanylyl Cyclase | 984 | ||
Receptor Tyrosine Kinases | 984 | ||
Tyrosine Kinase–Associated Receptors | 984 | ||
Amine Hormones | 984 | ||
Amine hormones are made from tyrosine and tryptophan | 984 | ||
Amine hormones act via surface receptors | 984 | ||
Steroid and Thyroid Hormones | 985 | ||
Cholesterol is the precursor for the steroid hormones: cortisol, aldosterone, estradiol, progesterone, and testosterone | 985 | ||
Steroid hormones bind to intracellular receptors that regulate gene transcription | 986 | ||
Thyroid hormones bind to intracellular receptors that regulate metabolic rate | 988 | ||
Steroid and thyroid hormones can also have nongenomic actions | 989 | ||
References | 989 | ||
References | 989.e1 | ||
Books and Reviews | 989.e1 | ||
Journal Articles | 989.e1 | ||
48 Endocrine Regulation of Growth and Body Mass | 990 | ||
Growth Hormone | 990 | ||
GH, secreted by somatotrophs in the anterior pituitary, is the principal endocrine regulator of growth | 990 | ||
GH is in a family of hormones with overlapping activity | 991 | ||
Somatotrophs secrete GH in pulses | 991 | ||
GH secretion is under hierarchical control by GH–releasing hormone and somatostatin | 992 | ||
GH-Releasing Hormone | 992 | ||
GHRH Receptor | 992 | ||
Ghrelin | 992 | ||
Ghrelin Receptor | 993 | ||
Somatostatin | 993 | ||
SS Receptor | 994 | ||
Both GH and IGF-1 negatively feed back on GH secretion by somatotrophs | 994 | ||
GH has short-term anti-insulin metabolic effects as well as long-term growth-promoting effects mediated by IGF-1 | 994 | ||
GH Receptor | 994 | ||
Short-Term Effects of GH | 994 | ||
Long-Term Effects of GH via IGF-1 | 994 | ||
Growth-Promoting Hormones | 996 | ||
IGF-1 is the principal mediator of the growth-promoting action of GH | 996 | ||
IGF-2 acts similarly to IGF-1 but is less dependent on GH | 996 | ||
Growth rate parallels plasma levels of IGF-1 except early and late in life | 998 | ||
Thyroid hormones, steroids, and insulin also promote growth | 999 | ||
Thyroid Hormones | 999 | ||
Sex Steroids | 999 | ||
Glucocorticoids | 999 | ||
Insulin | 999 | ||
The musculoskeletal system responds to growth stimuli of the GHRH–GH–IGF-1 axis | 1000 | ||
Regulation of Body Mass | 1000 | ||
The balance between energy intake and expenditure determines body mass | 1001 | ||
Energy expenditure comprises resting metabolic rate, activity-related energy expenditure, and diet-induced thermogenesis | 1001 | ||
Hypothalamic centers control the sensations of satiety and hunger | 1001 | ||
Leptin tells the brain how much fat is stored | 1001 | ||
Leptin and insulin are anorexigenic (i.e., satiety) signals for the hypothalamus | 1002 | ||
POMC Neurons | 1002 | ||
NPY/AgRP Neurons | 1002 | ||
Secondary Neurons | 1003 | ||
Ghrelin is an orexigenic signal for the hypothalamus | 1003 | ||
Plasma nutrient levels and enteric hormones are short-term factors that regulate feeding | 1003 | ||
References | 1005 | ||
References | 1005.e1 | ||
Books and Reviews | 1005.e1 | ||
Journal Articles | 1005.e1 | ||
49 The Thyroid Gland | 1006 | ||
Synthesis of Thyroid Hormones | 1006 | ||
T4 and T3, made by iodination of tyrosine residues on thyroglobulin, are stored as part of thyroglobulin molecules in thyroid follicles | 1006 | ||
Follicular cells take up iodinated thyroglobulin, hydrolyze it, and release T4 and T3 into the blood for binding to plasma proteins | 1007 | ||
Peripheral tissues deiodinate T4 to produce T3 | 1009 | ||
Action of Thyroid Hormones | 1010 | ||
Thyroid hormones act through nuclear receptors in target tissues | 1010 | ||
Thyroid hormones can also act by nongenomic pathways | 1011 | ||
Thyroid hormones increase basal metabolic rate by stimulating futile cycles of catabolism and anabolism | 1011 | ||
Carbohydrate Metabolism | 1012 | ||
Protein Metabolism | 1012 | ||
Lipid Metabolism | 1012 | ||
Na-K Pump Activity | 1012 | ||
Thermogenesis | 1013 | ||
Thyroid hormones are essential for normal growth and development | 1013 | ||
Hypothalamic-Pituitary-Thyroid Axis | 1014 | ||
TRH from the hypothalamus stimulates thyrotrophs of the anterior pituitary to secrete TSH, which stimulates T4/T3 synthesis | 1014 | ||
Thyrotropin-Releasing Hormone | 1014 | ||
TRH Receptor | 1014 | ||
Thyrotropin | 1016 | ||
TSH Receptor | 1016 | ||
T3 exerts negative feedback on TSH secretion | 1016 | ||
References | 1017 | ||
References | 1017.e2 | ||
Books and Reviews | 1017.e2 | ||
Journal Articles | 1017.e2 | ||
50 The Adrenal Gland | 1018 | ||
The Adrenal Cortex: Cortisol | 1018 | ||
Cortisol is the primary glucocorticoid hormone in humans | 1018 | ||
Target Tissues | 1018 | ||
Actions | 1018 | ||
The adrenal zona fasciculata converts cholesterol to cortisol | 1019 | ||
Cortisol binds to a cytoplasmic receptor that translocates to the nucleus and modulates transcription in multiple tissues | 1022 | ||
Corticotropin-releasing hormone from the hypothalamus stimulates anterior pituitary corticotrophs to secrete ACTH, which stimulates the adrenal cortex to synthesize and secrete cortisol | 1023 | ||
Corticotropin-Releasing Hormone | 1023 | ||
CRH Receptor | 1023 | ||
Arginine Vasopressin | 1023 | ||
Adrenocorticotropic Hormone | 1023 | ||
ACTH Receptor | 1023 | ||
Cortisol exerts negative feedback on CRH and ACTH secretion, whereas stress acts through higher CNS centers to stimulate the axis | 1025 | ||
Feedback to the Anterior Pituitary | 1025 | ||
Feedback to the Hypothalamus | 1025 | ||
Control by a Higher CNS Center | 1025 | ||
The Adrenal Cortex: Aldosterone | 1026 | ||
The mineralocorticoid aldosterone is the primary regulator of salt balance and extracellular volume | 1026 | ||
The glomerulosa cells of the adrenal cortex synthesize aldosterone from cholesterol via progesterone | 1026 | ||
Aldosterone stimulates Na+ reabsorption and K+ excretion by the renal tubule | 1027 | ||
Angiotensin II, K+, and ACTH all stimulate aldosterone secretion | 1027 | ||
Angiotensin II | 1028 | ||
Potassium | 1028 | ||
Adrenocorticotropic Hormone | 1028 | ||
Aldosterone exerts indirect negative feedback on the renin-angiotensin axis by increasing effective circulating volume and by lowering plasma [K+] | 1029 | ||
Renin-Angiotensin Axis | 1029 | ||
Potassium | 1029 | ||
Role of Aldosterone in Normal Physiology | 1030 | ||
Role of Aldosterone in Disease | 1030 | ||
The Adrenal Medulla | 1030 | ||
The adrenal medulla bridges the endocrine and sympathetic nervous systems | 1030 | ||
Only chromaffin cells of the adrenal medulla have the enzyme for epinephrine synthesis | 1030 | ||
Catecholamines bind to α and β adrenoceptors on the cell surface and act through heterotrimeric G proteins | 1033 | ||
The CNS-epinephrine axis provides integrated control of multiple functions | 1033 | ||
References | 1034 | ||
References | 1034.e1 | ||
Books and Reviews | 1034.e1 | ||
Journal Articles | 1034.e1 | ||
51 The Endocrine Pancreas | 1035 | ||
The islets of Langerhans are endocrine and paracrine tissue | 1035 | ||
Insulin | 1035 | ||
Insulin replenishes fuel reserves in muscle, liver, and adipose tissue | 1036 | ||
β cells synthesize and secrete insulin | 1037 | ||
The Insulin Gene | 1037 | ||
Insulin Synthesis | 1037 | ||
Secretion of Insulin, Proinsulin, and C Peptide | 1037 | ||
Glucose is the major regulator of insulin secretion | 1038 | ||
Metabolism of glucose by the β cell triggers insulin secretion | 1039 | ||
Neural and humoral factors modulate insulin secretion | 1041 | ||
Exercise | 1041 | ||
Feeding | 1041 | ||
The insulin receptor is a receptor tyrosine kinase | 1041 | ||
High levels of insulin lead to downregulation of insulin receptors | 1044 | ||
In liver, insulin promotes conversion of glucose to glycogen stores or to triacylglycerols | 1044 | ||
Glycogen Synthesis and Glycogenolysis | 1044 | ||
Glycolysis and Gluconeogenesis | 1045 | ||
Lipogenesis | 1047 | ||
Protein Metabolism | 1047 | ||
In muscle, insulin promotes the uptake of glucose and its storage as glycogen | 1047 | ||
In adipocytes, insulin promotes glucose uptake and conversion to TAGs for storage | 1047 | ||
Glucagon | 1050 | ||
Pancreatic α cells secrete glucagon in response to ingested protein | 1050 | ||
Pancreatic α Cells | 1050 | ||
Intestinal L Cells | 1051 | ||
Glucagon, acting through cAMP, promotes the synthesis of glucose by the liver | 1051 | ||
Glucagon promotes oxidation of fat in the liver, which can lead to ketogenesis | 1051 | ||
Somatostatin | 1053 | ||
Somatostatin inhibits the secretion of growth hormone, insulin, and other hormones | 1053 | ||
References | 1053 | ||
References | 1053.e1 | ||
Books and Reviews | 1053.e1 | ||
Journal Articles | 1053.e1 | ||
52 The Parathyroid Glands and Vitamin D | 1054 | ||
Calcium and Phosphate Balance | 1054 | ||
The gut, kidneys, and bone regulate calcium balance | 1054 | ||
The gut, kidneys, and bone also regulate phosphate balance | 1054 | ||
Physiology of Bone | 1056 | ||
Dense cortical bone and the more reticulated trabecular bone are the two major bone types | 1056 | ||
The extracellular matrix forms the nidus for the nucleation of hydroxyapatite crystals | 1057 | ||
Bone remodeling depends on the closely coupled activities of osteoblasts and osteoclasts | 1057 | ||
Parathyroid Hormone | 1058 | ||
Plasma Ca2+ regulates the synthesis and secretion of PTH | 1058 | ||
PTH Synthesis and Vitamin D | 1058 | ||
Processing of PTH | 1059 | ||
Metabolism of PTH | 1059 | ||
High plasma [Ca2+] inhibits the synthesis and release of PTH | 1060 | ||
The PTH receptor couples via G proteins to either adenylyl cyclase or phospholipase C | 1061 | ||
In the kidney, PTH promotes Ca2+ reabsorption, phosphate loss, and 1-hydroxylation of 25-hydroxyvitamin D | 1061 | ||
Stimulation of Ca2+ Reabsorption | 1062 | ||
Inhibition of Phosphate Reabsorption | 1062 | ||
Stimulation of the Last Step of Synthesis of 1,25- Dihydroxyvitamin D | 1062 | ||
In bone, PTH can promote net resorption or net deposition | 1063 | ||
Bone Resorption by Indirect Stimulation of Osteoclasts | 1063 | ||
Bone Resorption by Reduction in Bone Matrix | 1063 | ||
Bone Deposition | 1063 | ||
Vitamin D | 1063 | ||
The active form of vitamin D is its 1,25-dihydroxy metabolite | 1063 | ||
Vitamin D, by acting on the small intestine and kidney, raises plasma [Ca2+] and thus promotes bone mineralization | 1065 | ||
Small Intestine | 1065 | ||
Kidney | 1065 | ||
Bone | 1065 | ||
Calcium ingestion lowers—whereas phosphate ingestion raises—levels of both PTH and 1,25-dihydroxyvitamin D | 1067 | ||
Calcium Ingestion | 1067 | ||
Phosphate Ingestion | 1067 | ||
Calcitonin and Other Hormones | 1067 | ||
Calcitonin inhibits osteoclasts, but its effects are transitory | 1067 | ||
Sex steroid hormones promote bone deposition, whereas glucocorticoids promote resorption | 1068 | ||
PTHrP, encoded by a gene that is entirely distinct from that for PTH, can cause hypercalcemia in certain malignancies | 1069 | ||
References | 1069 | ||
References | 1069.e2 | ||
Books and Reviews | 1069.e2 | ||
Journal Articles | 1069.e2 | ||
IX The Reproductive System | 1071 | ||
53 Sexual Differentiation | 1072 | ||
Genetic Aspects of Sexual Differentiation | 1072 | ||
Meiosis occurs only in germ cells and gives rise to male and female gametes | 1072 | ||
Fertilization of an oocyte by an X- or Y-bearing sperm establishes the zygote’s genotypic sex | 1073 | ||
Genotypic sex determines differentiation of the indifferent gonad into either an ovary or a testis | 1075 | ||
The testis-determining gene is located on the Y chromosome | 1075 | ||
Endocrine and paracrine messengers modulate phenotypic differentiation | 1076 | ||
Differentiation of the Gonads | 1076 | ||
Primordial germ cells migrate from the yolk sac to the primordial gonad | 1076 | ||
The primitive testis develops from the medulla of the primordial gonad | 1078 | ||
The primitive ovary develops from the cortex of the primordial gonad | 1078 | ||
Development of the Accessory Sex Organs | 1078 | ||
The embryonic gonad determines the development of the internal genitalia and the external sexual phenotype | 1078 | ||
Embryos of both sexes have a double set of embryonic genital ducts | 1078 | ||
In males, the wolffian ducts become the epididymis, vas deferens, seminal vesicles, and ejaculatory duct | 1079 | ||
In females, the müllerian ducts become the fallopian tubes, the uterus, and the upper third of the vagina | 1080 | ||
In males, development of the wolffian ducts requires testosterone | 1080 | ||
In males, antimüllerian hormone causes regression of the müllerian ducts | 1080 | ||
Differentiation of the External Genitalia | 1081 | ||
The urogenital sinus develops into the urinary bladder, the urethra, and, in females, the vestibule of the vagina | 1081 | ||
The external genitalia of both sexes develop from common anlagen | 1083 | ||
Endocrine and Paracrine Control of Sexual Differentiation | 1084 | ||
The SRY gene triggers development of the testis, which makes the androgens and AMH necessary for male sexual differentiation | 1084 | ||
Testosterone Production | 1085 | ||
Androgen Receptor | 1085 | ||
DHT Formation | 1085 | ||
Antimüllerian Hormone | 1085 | ||
Androgens direct the male pattern of sexual differentiation of the internal ducts, the urogenital sinus, and the external genitalia | 1085 | ||
Differentiation of the Duct System | 1086 | ||
Differentiation of the Urogenital Sinus and External Genitalia | 1086 | ||
Androgens and estrogens influence sexual differentiation of the brain | 1086 | ||
Puberty | 1087 | ||
Puberty involves steroid hormones produced by the gonads and the adrenals | 1087 | ||
Hypothalamic gonadotropin-releasing hormone secretion controls puberty | 1088 | ||
Multiple factors control the timing of puberty | 1088 | ||
Androgens and estrogens influence secondary sex characteristics at puberty | 1088 | ||
Males | 1088 | ||
Females | 1090 | ||
The appearance of secondary sex characteristics at puberty completes sexual differentiation and development | 1091 | ||
References | 1091 | ||
References | 1091.e1 | ||
Books and Reviews | 1091.e1 | ||
Journal Articles | 1091.e1 | ||
54 The Male Reproductive System | 1092 | ||
Hypothalamic-Pituitary-Gonadal Axis | 1092 | ||
The hypothalamus secretes GnRH, which acts on gonadotrophs in the anterior pituitary | 1092 | ||
Under the control of GnRH, gonadotrophs in the anterior pituitary secrete LH and FSH | 1094 | ||
LH stimulates the Leydig cells of the testis to produce testosterone | 1095 | ||
FSH stimulates Sertoli cells to synthesize hormones that influence Leydig cells and spermatogenesis | 1095 | ||
The hypothalamic-pituitary-testicular axis is under feedback inhibition by testicular steroids and inhibins | 1096 | ||
Testosterone | 1097 | ||
Leydig cells convert cholesterol to testosterone | 1097 | ||
Adipose tissue, skin, and the adrenal cortex also produce testosterone and other androgens | 1097 | ||
Testosterone acts on target organs by binding to a nuclear receptor | 1099 | ||
Metabolism of testosterone occurs primarily in the liver and prostate | 1099 | ||
Biology of Spermatogenesis and Semen | 1100 | ||
Spermatogenesis includes mitotic divisions of spermatogonia, meiotic divisions of spermatocytes to spermatids, and maturation to spermatozoa N54-7 | 1100 | ||
The Sertoli cells support spermatogenesis | 1100 | ||
Sperm maturation occurs in the epididymis | 1102 | ||
Spermatozoa are the only independently motile cells in the human body | 1103 | ||
The accessory male sex glands—the seminal vesicles, prostate, and bulbourethral glands—produce the seminal plasma | 1103 | ||
Male Sex Act | 1104 | ||
The sympathetic and parasympathetic divisions of the autonomic nervous system control the male genital system | 1104 | ||
Sympathetic Division of the ANS | 1104 | ||
Parasympathetic Division of the ANS | 1104 | ||
Visceral Afferents | 1105 | ||
Erection is primarily under parasympathetic control | 1105 | ||
Parasympathetic Innervation | 1106 | ||
Sympathetic Innervation | 1106 | ||
Somatic Innervation | 1106 | ||
Afferent Innervation | 1106 | ||
Emission is primarily under sympathetic control | 1106 | ||
Motor Activity of the Duct System | 1107 | ||
Secretory Activity of the Accessory Glands | 1107 | ||
Ejaculation is under the control of a spinal reflex | 1107 | ||
References | 1107 | ||
References | 1107.e2 | ||
Books and Reviews | 1107.e2 | ||
Journal Articles | 1107.e2 | ||
55 The Female Reproductive System | 1108 | ||
Female reproductive organs include the ovaries and accessory sex organs | 1108 | ||
Reproductive function in the human female is cyclic | 1108 | ||
Hypothalamic-Pituitary-Gonadal Axis and Control of the Menstrual Cycle | 1110 | ||
The human menstrual cycle coordinates changes in both the ovary and endometrium | 1110 | ||
Follicular/Proliferative Phase | 1110 | ||
Ovulation | 1111 | ||
Luteal/Secretory Phase | 1111 | ||
Menses | 1111 | ||
The hypothalamic-pituitary-ovarian axis drives the menstrual cycle | 1111 | ||
Neurons in the hypothalamus release GnRH in a pulsatile fashion | 1111 | ||
GnRH stimulates gonadotrophs in the anterior pituitary to secrete FSH and LH | 1111 | ||
The ovarian steroids (estrogens and progestins) feed back on the hypothalamic-pituitary axis | 1112 | ||
Negative Feedback by Ovarian Steroids | 1112 | ||
Positive Feedback by Ovarian Steroids | 1113 | ||
Ovaries produce peptide hormones—inhibins, activins, and follistatins—that modulate FSH secretion | 1113 | ||
Negative Feedback by the Inhibins | 1114 | ||
Positive Feedback by the Activins | 1114 | ||
Modulation of gonadotropin secretion by positive and negative ovarian feedback produces the normal menstrual rhythm | 1115 | ||
Ovarian Steroids | 1116 | ||
Starting from cholesterol, the ovary synthesizes estradiol, the major estrogen, and progesterone, the major progestin | 1116 | ||
Estrogen biosynthesis requires two ovarian cells and two gonadotropins, whereas progestin synthesis requires only a single cell | 1117 | ||
Estrogens stimulate cellular proliferation and growth of sex organs and other tissues related to reproduction | 1119 | ||
The Ovarian Cycle: Folliculogenesis, Ovulation, and Formation of the Corpus Luteum | 1120 | ||
Female reproductive life span is determined by the number of primordial follicles established during fetal life | 1120 | ||
Primary Oocytes | 1120 | ||
Primordial Follicles | 1121 | ||
Primary Follicles | 1122 | ||
Secondary Follicles | 1122 | ||
Tertiary Follicles | 1122 | ||
Graafian Follicles | 1122 | ||
The oocyte grows and matures during folliculogenesis | 1122 | ||
FSH and LH stimulate the growth of a cohort of follicles | 1122 | ||
Each month, one follicle achieves dominance | 1123 | ||
Estradiol secretion by the dominant follicle triggers the LH surge and thus ovulation | 1123 | ||
After ovulation, theca and granulosa cells of the follicle differentiate into theca-lutein and granulosa-lutein cells of the corpus luteum | 1124 | ||
Growth and involution of the corpus luteum produce the rise and fall in estradiol and progesterone during the luteal phase | 1124 | ||
The Endometrial Cycle | 1124 | ||
The ovarian hormones drive the morphological and functional changes of the endometrium during the monthly cycle | 1124 | ||
The Menstrual Phase | 1124 | ||
The Proliferative Phase | 1124 | ||
The Secretory Phase | 1125 | ||
The effective implantation window is 3 to 4 days | 1126 | ||
Female Sex Act | 1126 | ||
The female sex response occurs in four distinct phases | 1126 | ||
Excitement | 1126 | ||
Plateau | 1127 | ||
Orgasm | 1127 | ||
Resolution | 1127 | ||
Both the sympathetic and the parasympathetic divisions control the female sex response | 1127 | ||
The female sex response facilitates sperm transport through the female reproductive tract | 1127 | ||
Menopause | 1127 | ||
Only a few functioning follicles remain in the ovaries of a menopausal woman | 1127 | ||
During menopause, levels of the ovarian steroids fall, whereas gonadotropin levels rise | 1128 | ||
References | 1128 | ||
References | 1128.e1 | ||
Books and Reviews | 1128.e1 | ||
Journal Articles | 1128.e1 | ||
56 Fertilization, Pregnancy, and Lactation | 1129 | ||
Transport of Gametes and Fertilization | 1129 | ||
Cilia and smooth muscle transport the egg and sperm within the female genital tract | 1129 | ||
The “capacitation” of the spermatozoa that occurs in the female genital tract enhances the ability of the sperm cell to fertilize the ovum | 1129 | ||
Fertilization begins as the sperm cell attaches to the zona pellucida and undergoes the acrosomal reaction, and it ends with the fusion of the male and female pronuclei | 1129 | ||
Implantation of the Developing Embryo | 1132 | ||
The presence of an embryo leads to decidualization of the endometrium | 1133 | ||
Uterine secretions nourish the preimplantation embryo, promote growth, and prepare it for implantation | 1133 | ||
The blastocyst secretes substances that facilitate implantation | 1133 | ||
During implantation, the blastocyst apposes itself to the endometrium, adheres to epithelial cells, and finally invades the stroma | 1134 | ||
Apposition | 1134 | ||
Adhesion | 1135 | ||
Invasion | 1136 | ||
Physiology of the Placenta | 1136 | ||
At the placenta, the space between the fetus’s chorionic villi and the mother’s endometrial wall contains a continuously renewed pool of extravasated maternal blood | 1136 | ||
Maternal Blood Flow | 1136 | ||
Fetal Blood Flow | 1137 | ||
Gases and other solutes move across the placenta | 1137 | ||
O2 and CO2 Transport | 1137 | ||
Other Solutes | 1138 | ||
The placenta makes a variety of peptide hormones, including hCG and human chorionic somatomammotropin | 1139 | ||
The Maternal-Placental-Fetal Unit | 1139 | ||
During pregnancy, progesterone and estrogens rise to levels that are substantially higher than their peaks in a normal cycle | 1139 | ||
After 8 weeks of gestation, the maternal-placental-fetal unit maintains high levels of progesterone and estrogens | 1140 | ||
Response of the Mother to Pregnancy | 1142 | ||
Both maternal cardiac output and blood volume increase during pregnancy | 1142 | ||
Increased levels of progesterone during pregnancy increase alveolar ventilation | 1143 | ||
Pregnancy increases the demand for dietary protein, iron, and folic acid | 1143 | ||
Less than one third of the total maternal weight gain during pregnancy represents the fetus | 1143 | ||
Parturition | 1144 | ||
Human birth usually occurs at around the 40th week of gestation | 1144 | ||
Parturition occurs in distinct stages, numbered 0 to 3 | 1144 | ||
Stage 0—Quiescence | 1144 | ||
Stage 1—Transformation/Activation | 1144 | ||
Stage 2—Active Labor | 1144 | ||
Stage 3—Involution | 1144 | ||
Reciprocal decreases in progesterone receptors and increases in estrogen receptors are critical for the onset of labor | 1144 | ||
Signals from the fetus may initiate labor | 1145 | ||
PGs initiate uterine contractions, and both PGs and OT sustain labor | 1145 | ||
Prostaglandins | 1145 | ||
Oxytocin | 1145 | ||
Relaxin | 1146 | ||
Mechanical Factors | 1146 | ||
Positive Feedback | 1146 | ||
Lactation | 1146 | ||
The epithelial alveolar cells of the mammary gland secrete the complex mixture of sugars, proteins, lipids, and other substances that constitute milk | 1146 | ||
PRL is essential for milk production, and suckling is a powerful stimulus for PRL secretion | 1148 | ||
OT and psychic stimuli initiate milk ejection (“let-down”) | 1150 | ||
Suckling inhibits the ovarian cycle | 1150 | ||
References | 1150 | ||
References | 1150.e1 | ||
Books and Reviews | 1150.e1 | ||
Journal Articles | 1150.e1 | ||
57 Fetal and Neonatal Physiology | 1151 | ||
Biology of Fetal Growth | 1151 | ||
Two distinct circulations—fetoplacental and uteroplacental—underlie the transfer of gases and nutrients | 1151 | ||
Growth occurs by hyperplasia and hypertrophy | 1151 | ||
Growth depends primarily on genetic factors during the first half of gestation and on epigenetic factors thereafter | 1152 | ||
Increases in placental mass parallel periods of rapid fetal growth | 1152 | ||
Insulin, the insulin-like growth factors, and thyroxine stimulate fetal growth | 1153 | ||
Glucocorticoids and Insulin | 1153 | ||
Insulin-Like Growth Factors | 1154 | ||
Epidermal Growth Factor | 1154 | ||
Thyroid Hormones | 1154 | ||
Peptide Hormones | 1154 | ||
Many fetal tissues produce red blood cells early in gestation | 1154 | ||
The fetal gastrointestinal and urinary systems excrete products into the amniotic fluid by midpregnancy | 1154 | ||
A surge in protein synthesis, with an increase in muscle mass, is a major factor in the rapid fetal weight gain during the third trimester | 1155 | ||
Fetal lipid stores increase rapidly during the third trimester | 1155 | ||
Development and Maturation of the Cardiopulmonary System | 1155 | ||
Fetal lungs develop by repetitive branching of both bronchial and pulmonary arterial trees | 1155 | ||
An increase in cortisol, with other hormones, triggers surfactant production in the third trimester | 1156 | ||
Fetal respiratory movements begin near the end of the first trimester but wane just before birth | 1157 | ||
The fetal circulation has four unique pathways—placenta, ductus venosus, foramen ovale, and ductus arteriosus—to facilitate gas and nutrient exchange | 1157 | ||
Placenta | 1158 | ||
Ductus Venosus | 1158 | ||
Foramen Ovale | 1158 | ||
Ductus Arteriosus | 1158 | ||
Cardiopulmonary Adjustments at Birth | 1159 | ||
Loss of the placental circulation requires the newborn to breathe on its own | 1159 | ||
Mild hypoxia and hypercapnia, as well as tactile stimuli and cold skin, trigger the first breath | 1159 | ||
At birth, removal of the placenta increases systemic vascular resistance, whereas lung expansion decreases pulmonary vascular resistance | 1162 | ||
Removal of the Placental Circulation | 1162 | ||
Increase in Pulmonary Blood Flow | 1162 | ||
Closure of the ductus venosus within the first days of life forces portal blood to perfuse the liver | 1162 | ||
Closure of the foramen ovale occurs as left atrial pressure begins to exceed right atrial pressure | 1162 | ||
Closure of the ductus arteriosus completes the separation between the pulmonary and systemic circulations | 1163 | ||
Neonatal Physiology | 1164 | ||
Although the newborn is prone to hypothermia, nonshivering thermogenesis in brown fat helps to keep the neonate warm | 1164 | ||
The neonate mobilizes glucose and FAs soon after delivery | 1166 | ||
Carbohydrate Metabolism | 1166 | ||
Fat Metabolism | 1166 | ||
Metabolic Rate | 1166 | ||
Breast milk from a mother with a balanced diet satisfies all of the infant’s nutritional requirements during the first several months of life | 1166 | ||
The neonate is at special risk of developing fluid and acid-base imbalances | 1167 | ||
Humoral and cellular immune responses begin at early stages of development in the fetus | 1167 | ||
Fetus | 1167 | ||
Neonate | 1167 | ||
In premature newborns, immaturity of organ systems and fragility of homeostatic mechanisms exacerbate postnatal risks | 1168 | ||
References | 1168 | ||
References | 1168.e2 | ||
Books and Reviews | 1168.e2 | ||
Journal Articles | 1168.e2 | ||
X Physiology of Everyday Life | 1169 | ||
58 Metabolism | 1170 | ||
Forms of Energy | 1170 | ||
Energy Balance | 1172 | ||
Energy input to the body is the sum of energy output and storage | 1172 | ||
The inefficiency of chemical reactions leads to loss of the energy available for metabolic processes | 1173 | ||
Free energy, conserved as high-energy bonds in ATP, provides the energy for cellular functions | 1174 | ||
Energy Interconversion From Cycling between 6-Carbon and 3-Carbon Molecules | 1174 | ||
Glycolysis converts the 6-carbon glucose molecule to two 3-carbon pyruvate molecules | 1174 | ||
Gluconeogenesis converts nonhexose precursors to the 6-carbon glucose molecule | 1176 | ||
Reciprocal regulation of glycolysis and gluconeogenesis minimizes futile cycling | 1178 | ||
Allosteric Regulation | 1178 | ||
Transcriptional Regulation | 1178 | ||
Cells can convert glucose or amino acids into FAs | 1178 | ||
The body permits only certain energy interconversions | 1179 | ||
Energy Capture (Anabolism) | 1179 | ||
After a carbohydrate meal, the body burns some ingested glucose and incorporates the rest into glycogen or TAGs | 1179 | ||
Liver | 1179 | ||
Muscle | 1181 | ||
Adipose Tissue | 1181 | ||
After a protein meal, the body burns some ingested amino acids and incorporates the rest into proteins | 1181 | ||
After a fatty meal, the body burns some ingested FAs and incorporates the rest into TAGs | 1182 | ||
Energy Liberation (Catabolism) | 1182 | ||
The first step in energy catabolism is to break down glycogen or TAGs to simpler compounds | 1182 | ||
Skeletal Muscle | 1182 | ||
Liver | 1182 | ||
Adipocytes | 1182 | ||
The second step in TAG catabolism is β-oxidation of FAs | 1183 | ||
The final common steps in oxidizing carbohydrates, TAGs, and proteins to CO2 are the citric acid cycle and oxidative phosphorylation | 1185 | ||
Citric Acid Cycle | 1185 | ||
Oxidative Phosphorylation | 1185 | ||
Ketogenesis | 1185 | ||
Oxidizing different fuels yields similar amounts of energy per unit O2 consumed | 1187 | ||
Integrative Metabolism During Fasting | 1188 | ||
During an overnight fast, glycogenolysis and gluconeogenesis maintain plasma glucose levels | 1189 | ||
Requirement for Glucose | 1189 | ||
Gluconeogenesis versus Glycogenolysis | 1189 | ||
Gluconeogenesis: The Cori Cycle | 1189 | ||
Gluconeogenesis: The Glucose-Alanine Cycle | 1189 | ||
Lipolysis | 1190 | ||
Starvation beyond an overnight fast enhances gluconeogenesis and lipolysis | 1190 | ||
Enhanced Gluconeogenesis | 1190 | ||
Enhanced Lipolysis | 1191 | ||
Prolonged starvation moderates proteolysis but accelerates lipolysis, thereby releasing ketone bodies | 1191 | ||
Decreased Proteolysis | 1191 | ||
Decreased Hepatic Gluconeogenesis | 1191 | ||
Increased Renal Gluconeogenesis | 1191 | ||
Increased Lipolysis and Ketogenesis | 1191 | ||
References | 1192 | ||
References | 1192.e1 | ||
Books and Reviews | 1192.e1 | ||
Journal Articles | 1192.e1 | ||
59 Regulation of Body Temperature | 1193 | ||
Heat and Temperature: Advantages of Homeothermy | 1193 | ||
Homeotherms maintain their activities over a wide range of environmental temperatures | 1193 | ||
Body core temperature depends on time of day, physical activity, time in the menstrual cycle, and age | 1193 | ||
The body’s rate of heat production can vary from ~70 kcal/hr at rest to 600 kcal/hr during exercise | 1194 | ||
Modes of Heat Transfer | 1194 | ||
Maintaining a relatively constant body temperature requires a fine balance between heat production and heat losses | 1194 | ||
Heat moves from the body core to the skin, primarily by convection | 1195 | ||
Heat moves from the skin to the environment by radiation, conduction, convection, and evaporation | 1196 | ||
Radiation | 1196 | ||
Conduction | 1196 | ||
Convection | 1197 | ||
Evaporation | 1197 | ||
When heat gain exceeds heat loss, body core temperature rises | 1197 | ||
Clothing insulates the body from the environment and limits heat transfer from the body to the environment | 1198 | ||
Active Regulation of Body Temperature by the Central Nervous System | 1198 | ||
Thermoreceptors in the skin and temperature-sensitive neurons in the hypothalamus respond to changes in their local temperature | 1198 | ||
Skin Thermoreceptors | 1198 | ||
Hypothalamic Temperature-Sensitive Neurons | 1199 | ||
The CNS thermoregulatory network integrates thermal information and directs changes in efferent activity to modify rates of heat transfer and production | 1200 | ||
Thermal effectors include behavior, cutaneous circulation, sweat glands, and skeletal muscles responsible for shivering | 1200 | ||
Hypothermia, Hyperthermia, and Fever | 1201 | ||
Hypothermia or hyperthermia occurs when heat transfer to or from the environment overwhelms the body’s thermoregulatory capacity | 1201 | ||
Exercise raises heat production, which is followed by a matching rise in heat loss, but at the cost of a steady-state hyperthermia of exercise | 1202 | ||
Fever is a regulated hyperthermia | 1202 | ||
References | 1203 | ||
References | 1203.e2 | ||
Books and Reviews | 1203.e2 | ||
Journal Articles | 1203.e2 | ||
60 Exercise Physiology and Sports Science | 1204 | ||
Motor Units and Muscle Function | 1204 | ||
The motor unit is the functional element of muscle contraction | 1204 | ||
Muscle force rises with the recruitment of motor units and an increase in their firing frequency | 1204 | ||
Compared with type I motor units, type II units are faster and stronger but more fatigable | 1205 | ||
As external forces stretch muscle, series elastic elements contribute a larger fraction of total tension | 1206 | ||
The action of a muscle depends on the axis of its fibers and its origin and insertion on the skeleton | 1207 | ||
Fluid and energetically efficient movements require learning | 1207 | ||
Strength versus endurance training differentially alters the properties of motor units N60-3 | 1208 | ||
Conversion of Chemical Energy to Mechanical Work | 1208 | ||
ATP and PCr provide immediate but limited energy | 1208 | ||
Anaerobic glycolysis provides a rapid but self-limited source of ATP | 1209 | ||
Oxidation of glucose, lactate, and fatty acids provides a slower but long-term source of ATP | 1209 | ||
Oxidation of Nonmuscle Glucose | 1209 | ||
Oxidation of Lactate | 1211 | ||
Gluconeogenesis | 1211 | ||
Oxidation of Nonmuscle Lipid | 1211 | ||
Choice of Fuel Sources | 1211 | ||
Muscle Fatigue | 1212 | ||
Fatigued muscle produces less force and has a reduced velocity of shortening | 1212 | ||
Changes in the CNS produce central fatigue | 1212 | ||
Impaired excitability and impaired Ca2+ release can produce peripheral fatigue | 1212 | ||
High-Frequency Fatigue | 1212 | ||
Low-Frequency Fatigue | 1212 | ||
Fatigue can result from ATP depletion, lactic acid accumulation, and glycogen depletion | 1213 | ||
ATP Depletion | 1213 | ||
Lactic Acid Accumulation | 1213 | ||
Glycogen Depletion | 1213 | ||
Determinants of Maximal O2 Uptake and Consumption | 1213 | ||
Maximal O2 uptake by the lungs can exceed resting O2 uptake by more than 20-fold | 1213 | ||
O2 uptake by muscle is the product of muscle blood flow and O2 extraction | 1214 | ||
O2 delivery by the cardiovascular system is the limiting step for maximal O2 utilization | 1214 | ||
Limited O2 Uptake by the Lungs | 1214 | ||
Limited O2 Delivery by the Cardiovascular System | 1214 | ||
Limited O2 Extraction by Muscle | 1215 | ||
Effective circulating volume takes priority over cutaneous blood flow for thermoregulation | 1215 | ||
Sweating | 1215 | ||
Eccrine, but not apocrine, sweat glands contribute to temperature regulation | 1215 | ||
Eccrine sweat glands are tubules comprising a secretory coiled gland and a reabsorptive duct | 1216 | ||
Secretion by Coil Cells | 1218 | ||
Reabsorption by Duct Cells | 1218 | ||
The NaCl content of sweat increases with the rate of secretion but decreases with acclimatization to heat | 1218 | ||
Flow Dependence | 1218 | ||
Cystic Fibrosis | 1218 | ||
Replenishment | 1218 | ||
Acclimatization | 1219 | ||
The hyperthermia of exercise stimulates eccrine sweat glands | 1219 | ||
Endurance (Aerobic) Training | 1219 | ||
Aerobic training requires regular periods of stress and recovery | 1219 | ||
Aerobic training increases maximal O2 delivery by increasing plasma volume and maximal cardiac output | 1219 | ||
Maximizing Arterial O2 Content | 1219 | ||
Maximizing Cardiac Output | 1220 | ||
Aerobic training enhances O2 diffusion into muscle | 1220 | ||
Aerobic training increases mitochondrial content | 1220 | ||
References | 1222 | ||
References | 1222.e1 | ||
Books and Reviews | 1222.e1 | ||
Journal Articles | 1222.e1 | ||
61 Environmental Physiology | 1223 | ||
The Environment | 1223 | ||
Voluntary feedback control mechanisms can modulate the many layers of our external environment | 1223 | ||
Environmental temperature provides conscious clues for triggering voluntary feedback mechanisms | 1224 | ||
Room ventilation should maintain , , and levels of toxic substances within acceptable limits | 1224 | ||
Acceptable Limits for and | 1224 | ||
Measuring Room Ventilation | 1224 | ||
Carbon Monoxide | 1224 | ||
Threshold Limit Values and Biological Exposure Indices | 1225 | ||
Tissues must resist the G force produced by gravity and other mechanisms of acceleration | 1225 | ||
The partial pressures of gases—other than water—inside the body depend on Pb | 1225 | ||
Diving Physiology | 1225 | ||
Immersion raises Pb, thereby compressing gases in the lungs | 1225 | ||
SCUBA divers breathe compressed air to maintain normal lung expansion | 1226 | ||
Increased alveolar can cause narcosis | 1227 | ||
Increased alveolar can lead to O2 toxicity | 1227 | ||
Using helium to replace inspired N2 and O2 avoids nitrogen narcosis and O2 toxicity | 1228 | ||
After an extended dive, one must decompress slowly to avoid decompression illness | 1229 | ||
High-Altitude Physiology | 1230 | ||
Pb and ambient on top of Mount Everest are approximately one third of their values at sea level | 1230 | ||
Everest Base Camp | 1230 | ||
Peak of Mount Everest | 1230 | ||
Air Travel | 1230 | ||
Up to modest altitudes, arterial O2 content falls relatively less than Pb due to the shape of the Hb-O2 dissociation curve | 1230 | ||
During the first few days at altitude, compensatory adjustments to hypoxemia include tachycardia and hyperventilation | 1231 | ||
Long-term adaptations to altitude include increases in hematocrit, pulmonary diffusing capacity, capillarity, and oxidative enzymes | 1231 | ||
Hematocrit | 1231 | ||
Pulmonary Diffusing Capacity | 1232 | ||
Capillary Density | 1232 | ||
Oxidative Enzymes | 1232 | ||
High altitude causes mild symptoms in most people and acute or chronic mountain sickness in susceptible individuals | 1232 | ||
Symptoms of Hypoxia | 1232 | ||
Acute Mountain Sickness | 1232 | ||
Chronic Mountain Sickness | 1232 | ||
Flight and Space Physiology | 1232 | ||
Acceleration in one direction shifts the blood volume in the opposite direction | 1232 | ||
“Weightlessness” causes a cephalad shift of the blood volume and an increase in urine output | 1233 | ||
Space flight leads to motion sickness and to decreases in muscle and bone mass | 1233 | ||
Exercise partially overcomes the deconditioning of muscles during space flight | 1234 | ||
Return to earth requires special measures to maintain arterial blood pressure | 1234 | ||
References | 1234 | ||
References | 1234.e1 | ||
Books and Reviews | 1234.e1 | ||
Journal Articles | 1234.e1 | ||
62 The Physiology of Aging | 1235 | ||
Concepts in Aging | 1235 | ||
During the 20th century, the age structure of populations in developed nations shifted toward older individuals | 1235 | ||
The definition, occurrence, and measurement of aging are fundamental but controversial issues | 1235 | ||
Aging is an evolved trait | 1235 | ||
Human aging studies can be cross-sectional or longitudinal | 1237 | ||
Cross-Sectional Design | 1237 | ||
Longitudinal Design | 1237 | ||
Whether age-associated diseases are an integral part of aging remains controversial | 1237 | ||
Cellular and Molecular Mechanisms of Aging | 1238 | ||
Oxidative stress and related processes that damage macromolecules may have a causal role in aging | 1238 | ||
Reactive Oxygen Species | 1238 | ||
Glycation and Glycoxidation | 1239 | ||
Mitochondrial Damage | 1239 | ||
Somatic Mutations | 1239 | ||
Inadequacy of repair processes may contribute to the aging phenotype | 1240 | ||
DNA Repair | 1240 | ||
Protein Homeostasis | 1240 | ||
Autophagy | 1240 | ||
Dysfunction of the homeostasis of cell number may be a major factor in aging | 1240 | ||
Limitations in Cell Division | 1240 | ||
Cell Removal | 1241 | ||
Aging of the Human Physiological Systems | 1242 | ||
Aging people lose height and lean body mass but gain and redistribute fat | 1243 | ||
Aging thins the skin and causes the musculoskeletal system to become weak, brittle, and stiff | 1243 | ||
Skin | 1243 | ||
Skeletal Muscle | 1243 | ||
Bone | 1243 | ||
Synovial Joints | 1243 | ||
The healthy elderly experience deficits in sensory transduction and speed of central processing | 1244 | ||
Sensory Functions | 1244 | ||
Motor Functions | 1244 | ||
Cognitive Functions | 1244 | ||
Aging causes decreased arterial compliance and increased ventilation-perfusion mismatching | 1244 | ||
Cardiovascular Function | 1244 | ||
Pulmonary Function | 1244 | ||
Exercise | 1244 | ||
Glomerular filtration rate falls with age in many but not all people | 1245 | ||
Aging has only minor effects on gastrointestinal function | 1245 | ||
Aging causes modest declines in most endocrine functions | 1245 | ||
Insulin | 1245 | ||
Growth Hormone and IGF-1 | 1245 | ||
Adrenal Steroids | 1245 | ||
Thyroid Hormones | 1245 | ||
Parathyroid Hormone | 1245 | ||
Gonadal Hormones | 1245 | ||
Aging Slowly | 1246 | ||
Caloric restriction slows aging and extends life in several species, including some mammals | 1246 | ||
Genetic alterations can extend life in several species | 1246 | ||
Proposed interventions to slow aging and extend human life are controversial | 1247 | ||
References | 1247 | ||
References | 1247.e1 | ||
Books and Reviews | 1247.e1 | ||
Journal Articles | 1247.e1 | ||
Index | 1249 | ||
Numbers | 1249 | ||
A | 1249 | ||
B | 1252 | ||
C | 1255 | ||
D | 1259 | ||
E | 1261 | ||
F | 1264 | ||
G | 1266 | ||
H | 1269 | ||
I | 1271 | ||
J | 1272 | ||
K | 1273 | ||
L | 1273 | ||
M | 1274 | ||
N | 1277 | ||
O | 1279 | ||
P | 1280 | ||
Q | 1286 | ||
R | 1286 | ||
S | 1288 | ||
T | 1292 | ||
U | 1295 | ||
V | 1295 | ||
W | 1296 | ||
X | 1296 | ||
Y | 1296 | ||
Z | 1296 |